Trigonal Bipyramidal: The Only Guide You'll Ever Need!
Understanding molecular geometry is paramount in chemistry, and trigonal bipyramidal is a fundamental concept within this domain. Valence Shell Electron Pair Repulsion (VSEPR) theory predicts the trigonal bipyramidal shape for molecules with five electron domains around a central atom. Inorganic chemists frequently encounter this geometry in compounds exhibiting intriguing properties. Furthermore, the trigonal bipyramidal structure significantly influences a molecule's polarity and reactivity, aspects meticulously studied at institutions like the California Institute of Technology.
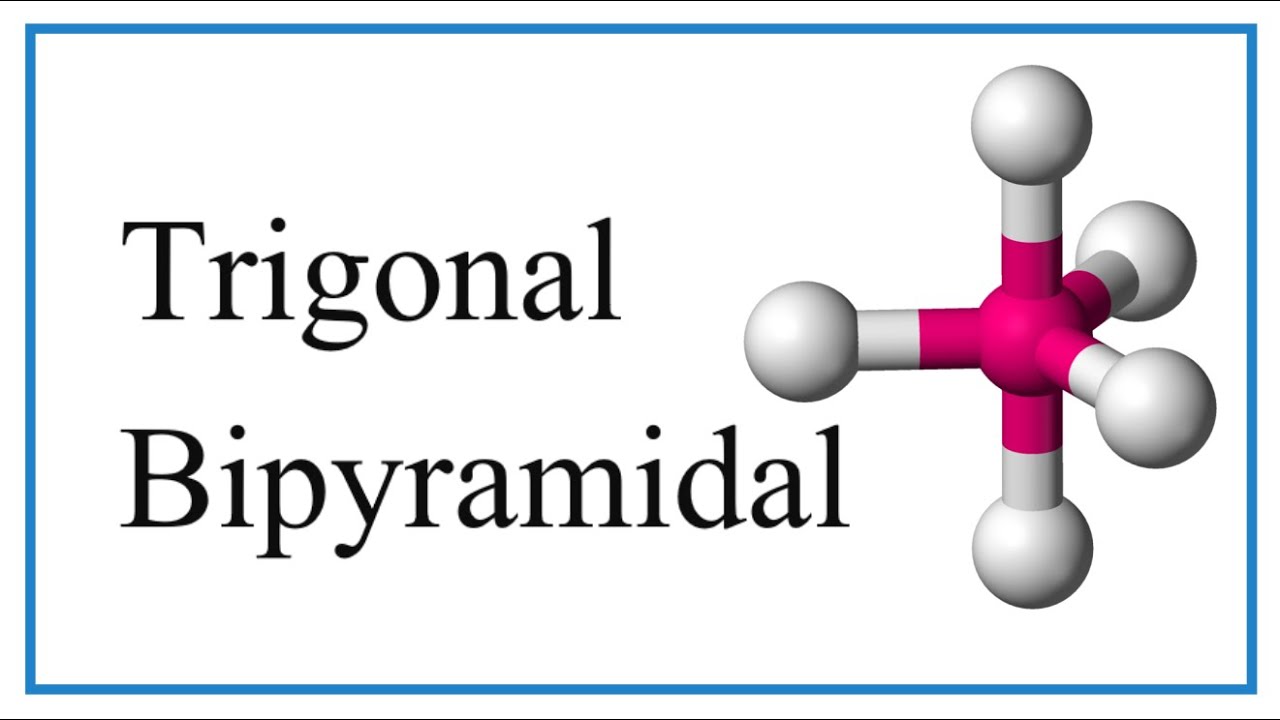
Image taken from the YouTube channel Wayne Breslyn (Dr. B.) , from the video titled Trigonal Bipyramidal Molecular Geometry/Shape and Bond Angles .
Molecular geometry, the three-dimensional arrangement of atoms within a molecule, dictates a substance’s physical and chemical behavior. This arrangement influences everything from a molecule's polarity and reactivity to its interactions with other molecules. Understanding these shapes is therefore fundamental to grasping chemical properties and predicting molecular behavior.
One particularly interesting and important molecular geometry is the trigonal bipyramidal shape.
This guide will delve into the specifics of this geometry, illuminating its characteristics, the factors that influence it, and its manifestation in real-world molecules. We will explore the underlying principles that govern this unique shape, providing a comprehensive understanding of its significance in the broader field of chemistry.
Molecular Geometry: A Foundation of Chemical Understanding
At its core, molecular geometry reveals how atoms organize themselves in space to form molecules. This arrangement is not arbitrary; it is dictated by the electronic structure of the atoms involved and the forces that govern their interactions.
Different geometries lead to different molecular properties.
For example, a linear molecule will behave differently than a bent molecule, even if they are composed of the same atoms.
Understanding molecular geometry allows chemists to predict and explain a wide range of phenomena, including reaction mechanisms, intermolecular forces, and the physical properties of materials.
Defining the Trigonal Bipyramidal Shape
The term "trigonal bipyramidal" describes a specific arrangement of five atoms or groups of atoms (ligands) around a central atom.
Imagine a central atom positioned in the middle. Three ligands form a triangle around the central atom's "equator," creating a trigonal plane.
Then, picture two more ligands positioned above and below the central atom, along an axis perpendicular to the trigonal plane.
These are at the "poles" of our central atom.
This overall arrangement results in two pyramids sharing a common triangular base, hence the name "trigonal bipyramidal."
Key characteristics include the presence of both axial and equatorial positions (which we will discuss in detail later) and specific bond angles that define the shape. This geometry is crucial in understanding the behavior of molecules like phosphorus pentachloride (PCl5) and related compounds.
Purpose and Scope of this Guide
This guide is designed to provide a comprehensive overview of the trigonal bipyramidal molecular geometry.
Our journey will begin with the underlying principles of VSEPR theory, which predicts molecular shapes by minimizing electron pair repulsion.
We will then explore the unique aspects of the trigonal bipyramidal arrangement, including the distinction between axial and equatorial positions and the influence of lone pairs of electrons.
Finally, we will examine real-world examples of molecules exhibiting this geometry and discuss the dynamic process of Berry pseudorotation.
By the end of this guide, you will have a strong understanding of the trigonal bipyramidal shape, its underlying principles, and its significance in the realm of molecular chemistry.
Molecular geometry allows us to visualize and understand the spatial arrangement of atoms within a molecule. But what principles guide this arrangement? What rules dictate whether a molecule adopts a linear, tetrahedral, or, in our case, a trigonal bipyramidal shape? The answer lies in a powerful predictive tool known as Valence Shell Electron Pair Repulsion (VSEPR) theory. This theory provides a framework for understanding and predicting molecular shapes based on the fundamental principle of minimizing repulsive forces between electron pairs.
VSEPR Theory: The Foundation of Molecular Shape Prediction
VSEPR theory serves as a cornerstone in predicting molecular shapes by focusing on the arrangement of electron pairs around a central atom. It operates on the principle that electron pairs, whether in bonds or lone pairs, will arrange themselves to minimize repulsion.
This minimization of repulsion dictates the geometry a molecule will adopt. By understanding VSEPR theory, chemists can effectively predict and explain the three-dimensional structures of molecules.
Minimizing Electron Pair Repulsion: The Guiding Principle
The central tenet of VSEPR theory is the minimization of electron pair repulsion. Electron pairs, being negatively charged, naturally repel each other. This repulsion forces them to arrange themselves as far apart as possible in three-dimensional space.
This spatial arrangement directly influences the molecule's shape. Different arrangements result in different geometries, each characterized by specific bond angles and overall symmetry.
VSEPR theory prioritizes reducing electron-electron repulsion as the driving force for molecular geometry. The goal is to maximize the distance between regions of electron density surrounding the central atom.
Applying VSEPR Theory to the Trigonal Bipyramidal Arrangement
The trigonal bipyramidal geometry presents a unique scenario for VSEPR theory due to its five electron domains around a central atom. This arrangement necessitates a specific spatial distribution to minimize electron pair repulsion.
Five Electron Domains: Axial and Equatorial Positions
In a trigonal bipyramidal arrangement, the five electron domains are distributed in two distinct positions: axial and equatorial. Three electron domains occupy the equatorial plane, forming a triangle around the central atom. The remaining two electron domains are positioned axially, above and below the equatorial plane.
The arrangement of these five electron domains around the central atom is crucial for minimizing repulsion.
The spatial distribution influences the bond angles and overall stability of the molecule. Understanding the interplay between axial and equatorial positions is essential for predicting molecular properties and reactivity.
The goal is to create a comprehensive and engaging section that thoroughly covers the distinctions between axial and equatorial positions, their spatial arrangement, and the significance of their unique bond angles within the trigonal bipyramidal geometry.
Axial vs. Equatorial: Defining Positions and Bond Angles
Understanding VSEPR theory provides a crucial framework for predicting molecular geometry. However, to truly grasp the nuances of the trigonal bipyramidal shape, we must delve into the specific spatial arrangements of atoms. This involves differentiating between axial and equatorial positions and appreciating how their distinct bond angles influence the overall properties of the molecule.
Distinguishing Axial and Equatorial Positions
The trigonal bipyramidal structure features two distinct types of positions around the central atom: axial and equatorial. Recognizing the difference between these positions is critical for predicting molecular behavior, especially when considering the placement of lone pairs.
Spatial Arrangement Around the Central Atom
Imagine the trigonal bipyramidal molecule as a combination of two simpler shapes: a trigonal plane and a linear arrangement. The equatorial positions form the trigonal plane, with three atoms arranged around the central atom at the corners of an equilateral triangle. This plane lies horizontally around the central atom.
In contrast, the axial positions lie above and below this equatorial plane, along a vertical axis that passes through the central atom. These two axial positions are directly opposite each other.
Bond Angles and Their Implications
The spatial arrangement of axial and equatorial positions dictates the bond angles within the molecule. These angles, in turn, have a significant impact on molecular properties, such as dipole moment and reactivity.
The 90° Angle Between Axial and Equatorial Bonds
Each axial atom forms a 90° angle with each of the three equatorial atoms. This means there are a total of six 90° interactions between the axial and equatorial substituents. The relative closeness of these interactions contributes significantly to steric crowding in this geometry.
The 120° Angle Between Equatorial Bonds
The three equatorial atoms are arranged in a trigonal planar arrangement. Hence they form a 120° angle with each other. This wider separation minimizes repulsion between the equatorial substituents. This arrangement leads to the equatorial positions being less sterically hindered compared to the axial positions.
Understanding these distinct bond angles is crucial for predicting how substituents, especially lone pairs, will arrange themselves around the central atom. This understanding is also critical in predicting the overall molecular shape and properties.
The angles we've just examined provide the foundation for understanding the ideal trigonal bipyramidal geometry. However, real molecules often deviate from this perfect shape. This deviation arises from the presence of lone pairs of electrons, which exert a significant influence on molecular geometry.
Lone Pair Influence: Distorting the Ideal Geometry
Lone pairs, unlike bonding pairs, are not constrained by the presence of another atom. This difference in constraint leads to a greater repulsive force exerted by lone pairs compared to bonding pairs. When a trigonal bipyramidal molecule contains lone pairs, the electron pair repulsion principles of VSEPR theory dictate that the molecule will distort to minimize these repulsions, leading to deviations from the ideal 90° and 120° bond angles.
The Repulsive Nature of Lone Pairs
Lone pairs of electrons, being held only by the central atom, are more diffuse and occupy more space around the central atom than bonding pairs.
This increased spatial requirement translates into a stronger repulsive force against other electron pairs, both bonding and non-bonding. This intensified repulsion is the key driver behind the distortion of the ideal trigonal bipyramidal geometry.
Equatorial Placement: Minimizing Repulsion
Given the heightened repulsive nature of lone pairs, their positioning within the trigonal bipyramidal structure is not arbitrary. VSEPR theory predicts, and experimental evidence confirms, that lone pairs preferentially occupy the equatorial positions.
But why is the equatorial position favored? To understand this, consider the interactions in each position:
An axial position has three neighboring electron pairs at 90° angles. Conversely, an equatorial position only has two neighboring electron pairs at 90° angles and two more distant neighbors at 120° angles.
The 90° interactions are far more repulsive than those at 120°. Hence, placing lone pairs in equatorial positions minimizes the overall repulsion within the molecule, leading to a more stable and energetically favorable configuration.
Distorted Shapes: See-Saw, T-Shaped, and Linear
The presence and positioning of lone pairs directly impact the overall shape of the molecule. By occupying equatorial positions, lone pairs effectively "push" the bonding pairs away, resulting in recognizable distortions from the ideal trigonal bipyramidal geometry. Several distinct shapes arise depending on the number of lone pairs present:
-
See-Saw Shape: When one lone pair occupies an equatorial position, the resulting shape resembles a see-saw or a distorted tetrahedron. The axial bonds bend away from the lone pair, leading to bond angles that are smaller than the ideal 90° and 120°. SF4 (Sulfur Tetrafluoride) is a classic example of a molecule exhibiting this geometry.
-
T-Shaped: With two lone pairs occupying two equatorial positions, the molecular shape becomes T-shaped. The two axial bonds are pushed away from the two lone pairs, forming a "T" with the central atom. ClF3 (Chlorine Trifluoride) exemplifies this geometry.
-
Linear: When three lone pairs occupy all three equatorial positions, the molecule adopts a linear shape. The two remaining bonding pairs are positioned directly opposite each other along the axial axis. XeF2 (Xenon Difluoride) demonstrates this linear arrangement.
These examples illustrate how lone pairs profoundly influence molecular shape. Understanding their preference for equatorial positions and their repulsive effects allows us to accurately predict and explain the observed geometries of various molecules.
The principles we've explored regarding lone pair placement and their repulsive forces now allow us to examine how these concepts manifest in real molecules. Phosphorus pentachloride, PCl5, stands as a quintessential example of a molecule adopting a trigonal bipyramidal geometry in the gas phase, showcasing the perfect arrangement – or deviations thereof – dictated by VSEPR theory.
PCl5 and Beyond: Real-World Examples of Trigonal Bipyramidal Molecules
Phosphorus pentachloride (PCl5) serves as a textbook example of a molecule exhibiting a trigonal bipyramidal geometry. Its structure provides a tangible representation of the principles discussed earlier. It allows us to understand how atoms arrange themselves to minimize electron repulsion.
Phosphorus Pentachloride (PCl5): A Detailed Look
The PCl5 molecule consists of a central phosphorus atom bonded to five chlorine atoms. These chlorine atoms occupy the five vertices of the trigonal bipyramid. This arrangement dictates the molecule's overall shape and properties.
The Central Phosphorus Atom
The phosphorus atom, in this case, is sp3d hybridized. This hybridization scheme results in five hybrid orbitals, which are directed towards the vertices of the trigonal bipyramid. Each of these hybrid orbitals overlaps with a p-orbital from a chlorine atom to form a sigma (σ) bond.
Axial and Equatorial Chlorine Atoms
As we've previously discussed, the trigonal bipyramidal geometry features two distinct positions: axial and equatorial. In PCl5, two chlorine atoms occupy the axial positions, situated directly above and below the central phosphorus atom. The remaining three chlorine atoms reside in the equatorial plane, forming a triangle around the phosphorus atom.
The axial P-Cl bonds are slightly longer (214 pm) than the equatorial P-Cl bonds (202 pm). This difference is attributed to the increased repulsion experienced by the axial chlorine atoms. They have three neighboring atoms at a 90° angle, unlike equatorial atoms which have only two.
Visualizing the Structure
Imagine a central phosphorus atom with three chlorine atoms arranged around it in a triangular shape. Now, picture two more chlorine atoms positioned above and below the phosphorus atom, perpendicular to the triangular plane. This mental image captures the essence of the PCl5 molecule's trigonal bipyramidal structure.
Ideally, molecular modeling software or textbooks provide clearer visual representations, showcasing the spatial arrangement of the atoms and the bond angles. These visuals solidify the understanding of this fundamental molecular geometry.
Beyond PCl5: Other Examples and Considerations
While PCl5 is a widely cited example, it's important to recognize that other molecules and ions also adopt a trigonal bipyramidal geometry. These include species like PF5 (phosphorus pentafluoride) and certain complex ions.
Furthermore, derivatives of PCl5 exist where one or more chlorine atoms are substituted with other ligands. These substitutions can lead to slight distortions in the geometry. They also influence the molecule's reactivity and physical properties. Examining these variations provides a deeper appreciation for the nuances of VSEPR theory. It also shows its predictive power in understanding molecular shapes.
Berry Pseudorotation: A Dynamic Molecular Dance
The arrangement of atoms in a trigonal bipyramidal molecule isn't as static as it might initially appear. Molecules are dynamic entities, constantly in motion, and PCl5 is no exception. It undergoes a fascinating process known as Berry pseudorotation, which significantly impacts its observable properties.
Understanding the Berry Mechanism
Berry pseudorotation, named after the scientist R. Stephen Berry, is a type of isomerization where a molecule seemingly rotates. What actually occurs is an intramolecular exchange of the axial and equatorial ligands (atoms or groups of atoms bound to the central atom).
Imagine the PCl5 molecule. During Berry pseudorotation, two axial chlorine atoms bend away from each other. The three equatorial chlorine atoms simultaneously move towards each other. This coordinated movement results in a square pyramidal transition state.
This transition state is where all five chlorine atoms are essentially equivalent. The molecule then proceeds to return to a trigonal bipyramidal structure. However, the chlorine atoms that were originally in axial positions now occupy equatorial positions, and vice versa.
This entire process occurs without breaking any chemical bonds. It's a continuous, fluid motion, making the molecule appear to rotate when observed over time.
Energy Considerations and the Rate of Exchange
Berry pseudorotation isn't spontaneous; it requires a certain amount of energy to overcome the activation barrier.
The energy needed for this process is relatively low. This means that at room temperature, Berry pseudorotation occurs rapidly in molecules like PCl5.
Spectroscopic techniques like Nuclear Magnetic Resonance (NMR) spectroscopy can be used to study this process. At low temperatures, the axial and equatorial chlorine atoms in PCl5 can be distinguished by NMR, because the pseudorotation is slowed.
However, as the temperature increases, the rate of pseudorotation increases. Eventually, the axial and equatorial chlorine atoms become indistinguishable on the NMR timescale. This is because they are rapidly interconverting.
Implications for Molecular Properties
The dynamic nature of Berry pseudorotation has important consequences for the observed properties of trigonal bipyramidal molecules.
One key implication is that it effectively averages the bond lengths. In a static trigonal bipyramidal structure, the axial and equatorial bonds have different lengths. However, due to the rapid exchange caused by Berry pseudorotation, the observed bond lengths appear to be the same when measured using techniques that average over time.
This dynamic behavior also impacts the molecule's reactivity. The ease with which ligands can exchange positions influences how the molecule interacts with other chemical species. This is particularly relevant in chemical reactions.
Video: Trigonal Bipyramidal: The Only Guide You'll Ever Need!
Frequently Asked Questions About Trigonal Bipyramidal Geometry
Hopefully, this guide provided a comprehensive overview of trigonal bipyramidal geometry. Here are some common questions and quick answers to further clarify the concepts.
What makes a molecule adopt a trigonal bipyramidal shape?
Molecules adopt a trigonal bipyramidal shape to minimize electron pair repulsion. This arrangement allows the five bonded atoms (or lone pairs) to be as far apart as possible, resulting in the lowest energy state for the molecule.
How are axial and equatorial positions different in a trigonal bipyramidal structure?
Axial positions are located above and below the central atom, while equatorial positions are arranged around the "equator" of the molecule. Axial positions experience more repulsion than equatorial positions due to having three neighboring groups at 90 degrees, whereas equatorial positions have two at 90 degrees.
Why do lone pairs prefer equatorial positions in a trigonal bipyramidal structure?
Lone pairs are more spatially demanding than bonding pairs. Placing them in equatorial positions minimizes their repulsion with the bonding pairs in a trigonal bipyramidal arrangement, leading to a more stable molecular geometry.
Can a molecule with six atoms around a central atom ever be trigonal bipyramidal?
No, a trigonal bipyramidal molecule will always have five atoms and zero or more lone pairs arranged around a central atom. If there are six atoms (or five atoms and a lone pair, etc.) around the central atom, then the molecular geometry is typically octahedral or another similar shape and never trigonal bipyramidal.