Tertiary Carbon: The Hidden Key to Organic Chemistry!
Organic chemistry relies heavily on understanding carbon structures. Steric hindrance, a crucial factor influencing reaction rates, is significantly affected by the presence of tertiary carbon atoms. These carbons, bonded to three other carbon atoms, play a vital role in determining the stability of carbocations, a concept often explored in depth using tools like NMR spectroscopy. Professor Pauling's work, alongside contributions from institutions such as MIT, highlights the importance of tertiary carbons in various organic reactions. Identifying and understanding tertiary carbon atoms is therefore essential for comprehending complex organic molecules and reaction mechanisms.
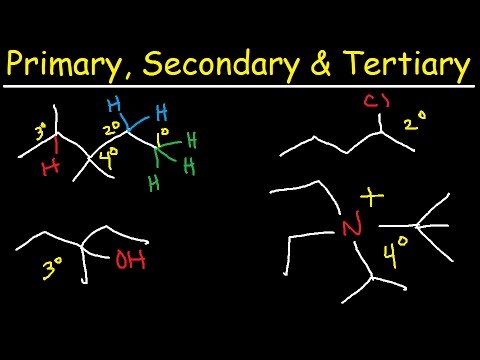
Image taken from the YouTube channel The Organic Chemistry Tutor , from the video titled Primary, Secondary, Tertiary, & Quarternary Hydrogen and Carbon Atoms .
Organic chemistry, at its core, is the study of carbon-containing compounds. Within this vast field, certain carbon atoms hold particular significance due to their unique structural environments and resulting reactivity. Among these, the tertiary carbon atom stands out as a pivotal player in shaping the course of numerous organic reactions.
Why Tertiary Carbons Matter
Consider the intricate dance of electrons during a chemical transformation. The tertiary carbon atom, bonded to three other carbon atoms, occupies a position of strategic importance. Its ability to stabilize developing charges, influence reaction pathways, and contribute to overall molecular stability makes it a key determinant in a wide array of chemical processes.
Understanding the behavior of tertiary carbon atoms is not merely an academic exercise. It's a necessity for anyone seeking to truly grasp the complexities of organic reactions.
Defining the Tertiary Carbon: A Structural Perspective
A tertiary carbon atom is defined as a carbon atom directly bonded to three other carbon atoms. This contrasts with primary carbons (bonded to one other carbon), secondary carbons (bonded to two other carbons), and quaternary carbons (bonded to four other carbons). The presence of three alkyl groups directly attached to the tertiary carbon creates a unique electronic and steric environment.
This specific bonding arrangement gives rise to several key properties, including enhanced stability of carbocations formed at that carbon and increased steric hindrance.
Thesis Statement: Exploring the Realm of Tertiary Carbons
This exploration delves into the fascinating world of tertiary carbon atoms. It will examine their structure, stability, reactivity, and the consequences of their unique position within organic molecules. By understanding the principles governing their behavior, we can gain a deeper appreciation for the intricate workings of organic chemistry.
This knowledge is crucial for predicting and controlling chemical reactions, designing new molecules, and ultimately, advancing our understanding of the world around us.
Organic chemistry, at its core, is the study of carbon-containing compounds. Within this vast field, certain carbon atoms hold particular significance due to their unique structural environments and resulting reactivity. Among these, the tertiary carbon atom stands out as a pivotal player in shaping the course of numerous organic reactions.
Why Tertiary Carbons Matter
Consider the intricate dance of electrons during a chemical transformation. The tertiary carbon atom, bonded to three other carbon atoms, occupies a position of strategic importance. Its ability to stabilize developing charges, influence reaction pathways, and contribute to overall molecular stability makes it a key determinant in a wide array of chemical processes.
Understanding the behavior of tertiary carbon atoms is not merely an academic exercise. It's a necessity for anyone seeking to truly grasp the complexities of organic reactions.
Thesis Statement: Exploring the Realm of Tertiary Carbons
This exploration delves into the fascinating world of tertiary carbon atoms. It will examine their structure, stability, and roles in various reaction mechanisms. But before diving into the intricacies of their reactivity, we must first establish a firm foundation by defining exactly what constitutes a tertiary carbon atom and how it differs from its counterparts.
Defining the Tertiary Carbon: Structure and Bonding
To fully appreciate the reactivity of a tertiary carbon, a rigorous definition of its structure is necessary. This foundational understanding provides the groundwork for analyzing its properties and role in chemical reactions.
The Tertiary Carbon Atom: A Precise Definition
A tertiary carbon atom is defined as a carbon atom that is directly bonded to three other carbon atoms. This means it forms three sigma bonds with neighboring carbon atoms and one sigma bond to a hydrogen atom or another substituent. It is this specific bonding arrangement that dictates its characteristic behavior in organic reactions.
This definition is crucial, as it sets the tertiary carbon apart from other types of carbon atoms in organic molecules. This structural difference profoundly influences its chemical properties.
Primary, Secondary, Tertiary, and Quaternary Carbons: A Comparative Overview
To truly understand the nature of a tertiary carbon atom, it's essential to compare it with its relatives: primary, secondary, and quaternary carbons. Each type differs in the number of carbon atoms to which it is directly bonded.
-
Primary (1°) Carbon: Bonded to only one other carbon atom.
-
Secondary (2°) Carbon: Bonded to two other carbon atoms.
-
Tertiary (3°) Carbon: Bonded to three other carbon atoms.
-
Quaternary (4°) Carbon: Bonded to four other carbon atoms.
These differences can be visually represented in a diagram. (Imagine a simple diagram here, showcasing each carbon type bonded to the appropriate number of other carbons).
It becomes clear that the tertiary carbon sits in a unique middle ground. It's not as exposed as a primary or secondary carbon, yet not as sterically crowded as a quaternary carbon.
This distinction plays a crucial role in determining its reactivity and stability.
Alkanes and the Tertiary Carbon: Illustrative Examples
Alkanes, simple hydrocarbons with single bonds, provide excellent examples of molecules containing tertiary carbon atoms. Consider the structure of 3-methylpentane.
(Imagine the structural formula of 3-methylpentane here).
The third carbon atom in the pentane chain is directly bonded to two other carbons in the chain and to the methyl group. This fits the definition of a tertiary carbon perfectly.
Another example is 2,3-dimethylbutane.
(Imagine the structural formula of 2,3-dimethylbutane here).
Here, carbons 2 and 3 are each bonded to three other carbons, making them tertiary carbons.
By visualizing these examples, the structural environment of the tertiary carbon becomes clear. It's a carbon atom strategically positioned within the molecule, ready to influence reaction pathways.
After all, understanding the very definition of a tertiary carbon atom is only the first step. The true power of this structural motif lies in its inherent stability, which profoundly influences reaction outcomes and dictates the behavior of molecules in countless chemical processes.
The Stability Advantage: Hyperconjugation and Inductive Effects
The enhanced stability of tertiary carbon atoms, particularly when bearing a positive charge as a carbocation, is a cornerstone of organic chemistry. This stability arises from a combination of electronic effects, primarily hyperconjugation and the inductive effect, both of which work to disperse the positive charge and lower the overall energy of the system.
Hyperconjugation: Sharing is Caring (Electrons)
Hyperconjugation is a stabilizing interaction that involves the overlap of a sigma (σ) bonding orbital with an adjacent empty or partially filled p-orbital. In the case of a tertiary carbocation, the positively charged carbon atom possesses an empty p-orbital.
The three alkyl groups attached to the tertiary carbon each have C-H σ bonds that can align with this empty p-orbital. This alignment allows the electrons in the σ bonds to delocalize into the empty p-orbital, effectively spreading the positive charge over a larger area of the molecule.
This delocalization stabilizes the carbocation.
The more alkyl groups attached to the carbocation center (as in a tertiary carbocation), the more σ bonds are available for hyperconjugation, leading to increased stability.
The Inductive Effect: A Helping Hand from Alkyl Groups
The inductive effect refers to the polarization of σ bonds due to differences in electronegativity between atoms. Alkyl groups are electron-donating groups, meaning they can push electron density towards the carbon atom to which they are attached.
In a tertiary carbocation, the three alkyl groups surrounding the positively charged carbon inductively donate electron density, helping to offset the positive charge and stabilize the ion.
While the inductive effect is generally weaker than hyperconjugation, it still contributes significantly to the overall stabilization of tertiary carbocations.
Tertiary vs. Secondary vs. Primary Carbocations: A Stability Hierarchy
The combination of hyperconjugation and the inductive effect results in a clear hierarchy of carbocation stability:
- Tertiary carbocations are the most stable.
- Secondary carbocations are intermediate in stability.
- Primary and methyl carbocations are the least stable.
Experimental data confirms this stability order. For instance, the activation energies for the formation of carbocations from alkyl halides decrease as the degree of substitution increases. This reflects the greater stability of the more substituted carbocations.
The relative stabilities can be estimated through computational chemistry methods.
Impact on Reaction Pathways: Steering the Course of Reactions
The stability of tertiary carbocations has a profound impact on the pathways of organic reactions. Reactions that proceed through carbocation intermediates, such as SN1 and E1 reactions, are strongly favored at tertiary carbon centers.
This is because the formation of a stable tertiary carbocation is a key step in these mechanisms. The increased stability of the carbocation lowers the activation energy for the reaction, making it more likely to occur.
Conversely, reactions that are disfavored by carbocation formation, such as SN2 reactions, are typically slower or nonexistent at tertiary carbons.
The preference for SN1 and E1 reactions at tertiary carbons is a direct consequence of the stability advantage conferred by hyperconjugation and the inductive effect. This principle is fundamental to understanding and predicting the outcomes of a wide range of organic reactions.
Tertiary Carbons in Action: SN1 and E1 Reaction Mechanisms
Having established the profound influence of hyperconjugation and inductive effects on the stability of tertiary carbocations, it’s time to explore how this enhanced stability translates into specific reaction pathways. Two prime examples are the SN1 (Substitution, Nucleophilic, Unimolecular) and E1 (Elimination, Unimolecular) reaction mechanisms, both of which are significantly influenced, and often favored, by the presence of a tertiary carbon atom.
The SN1 Reaction: A Tertiary Carbon's Playground
The SN1 reaction is a two-step process where the rate-determining step involves the formation of a carbocation intermediate.
This step is unimolecular, meaning the rate of the reaction depends only on the concentration of the substrate (the molecule containing the leaving group).
Tertiary alkyl halides excel in SN1 reactions due to the inherent stability of the tertiary carbocation that forms after the leaving group departs.
SN1 Mechanism Step-by-Step
-
Carbocation Formation (Rate-Determining Step): The reaction begins with the spontaneous departure of the leaving group (typically a halide ion) from the tertiary carbon atom. This generates a positively charged tertiary carbocation. The stability of this carbocation is the driving force behind the SN1 reaction at tertiary centers.
-
Nucleophilic Attack: The carbocation, now electron-deficient, is rapidly attacked by a nucleophile (an electron-rich species). Since the carbocation is planar (sp2 hybridized), the nucleophile can attack from either side of the molecule.
-
Protonation (If Necessary): If the nucleophile is neutral (e.g., an alcohol), the resulting product will be protonated. A subsequent deprotonation step, often by a solvent molecule, yields the final neutral substitution product.
The carbocation intermediate is planar, meaning the nucleophile can attack from either side.
This leads to a racemic mixture if the starting material was chiral.
Why Tertiary Carbons Favor SN1
The SN1 mechanism thrives with tertiary carbons for several reasons:
- Stable Carbocation Intermediate: As previously discussed, the tertiary carbocation is significantly more stable than primary or secondary carbocations due to hyperconjugation and inductive effects. This lowers the activation energy for the rate-determining step (carbocation formation), making the reaction faster.
- Reduced Steric Hindrance (Relative to SN2): While tertiary carbons are sterically hindered, this is less of a concern for SN1 reactions because the nucleophile attacks the relatively open carbocation intermediate after the bulky leaving group has already departed.
The E1 Reaction: A Competitive Pathway
The E1 reaction, like SN1, proceeds through a carbocation intermediate.
However, instead of nucleophilic attack, the carbocation loses a proton from a carbon atom adjacent to the positively charged carbon, forming a carbon-carbon double bond.
This results in the formation of an alkene.
E1 Mechanism Step-by-Step
- Carbocation Formation (Rate-Determining Step): This step is identical to the first step of the SN1 reaction. The leaving group departs, forming a tertiary carbocation.
- Deprotonation: A base (often the solvent) removes a proton from a carbon atom adjacent to the carbocation. The electrons from the C-H bond form a pi bond, generating an alkene.
E1 vs. SN1: The Battle for Dominance
E1 and SN1 reactions are often in competition, especially with tertiary substrates. Several factors influence which pathway will predominate:
- Temperature: Higher temperatures generally favor elimination (E1) due to the increased entropy associated with forming two molecules (alkene and acid) from one (alkyl halide).
- Base Strength: Stronger bases favor elimination (E2 – a different mechanism), while weaker bases typically favor SN1 or E1, depending on the substrate.
- Steric Hindrance: Increased steric hindrance around the carbocation favors elimination (E1) because it hinders the approach of a nucleophile for substitution (SN1).
In general, bulky bases are more likely to promote elimination because they have difficulty approaching the carbon atom for substitution.
Tertiary carbons are thus prone to undergoing E1 reactions, especially when heated or reacted with weaker bases.
Understanding these nuances is crucial for predicting and controlling reaction outcomes in organic synthesis.
Having explored the favorable aspects of tertiary carbons in unimolecular reactions, it’s crucial to acknowledge that their reactivity isn't universally advantageous. The very structural feature that contributes to carbocation stability – the presence of three bulky substituents – also introduces a significant constraint: steric hindrance.
The Flip Side: Steric Hindrance and its Consequences
While the electronic properties of tertiary carbon atoms often promote certain reactions, their spatial arrangement can significantly impede others. This section explores the concept of steric hindrance, its impact on reactions involving tertiary carbons, and how it can be strategically employed in organic synthesis.
Understanding Steric Hindrance
Steric hindrance arises from the spatial arrangement of atoms within a molecule. Specifically, it refers to the repulsion between electron clouds of atoms or groups that are close to each other.
This repulsion can impede the approach of a reactant to the reaction site, effectively slowing down or even preventing a reaction from occurring.
Steric Hindrance Around Tertiary Carbons
Tertiary carbon atoms, by definition, are bonded to three other carbon atoms. These carbon substituents, along with their attached hydrogen atoms or other groups, create a bulky environment around the central tertiary carbon.
This crowding can obstruct the approach of a reactant, making it difficult for it to interact with the tertiary carbon. The larger the substituents, the greater the steric hindrance.
SN2 Reactions: A Classic Case of Steric Inhibition
A prime example of how steric hindrance affects reactivity is the SN2 (Substitution, Nucleophilic, Bimolecular) reaction.
In an SN2 reaction, a nucleophile attacks the carbon atom bearing a leaving group, resulting in the simultaneous departure of the leaving group and the attachment of the nucleophile.
This reaction proceeds via a concerted mechanism, meaning it happens in a single step, with the nucleophile attacking from the backside of the carbon, opposite the leaving group.
Due to the backside attack mechanism, SN2 reactions are highly sensitive to steric hindrance. When the carbon atom is surrounded by bulky groups, like in a tertiary alkyl halide, the nucleophile finds it difficult to approach the carbon center.
Consequently, SN2 reactions are significantly disfavored at tertiary carbon atoms. The steric congestion makes it energetically unfavorable for the nucleophile to access the reaction site.
Exploiting Steric Hindrance for Selectivity
Despite its limitations, steric hindrance can be a valuable tool in organic synthesis. By strategically incorporating bulky groups into a molecule, chemists can control the regioselectivity (where a reaction occurs) and stereoselectivity (the stereochemical outcome of a reaction).
Protecting Groups
Bulky protecting groups are frequently used to temporarily block a specific reactive site on a molecule. This prevents unwanted reactions at that site while allowing reactions to proceed at other, less hindered positions.
Once the desired reaction has been carried out, the protecting group can be removed, revealing the original functional group.
Controlling Reaction Pathways
Steric hindrance can also be used to influence the pathway of a reaction. For example, in reactions where both substitution and elimination are possible, steric hindrance around a carbon atom can favor elimination over substitution.
This is because the transition state for elimination is often less sterically demanding than the transition state for substitution. By increasing the steric bulk around the reaction center, the elimination pathway can be selectively promoted.
In essence, while the stability conferred by tertiary carbons can be advantageous, the steric bulk they inherently possess can be strategically leveraged to achieve specific synthetic outcomes.
Having explored the favorable aspects of tertiary carbons in unimolecular reactions, it’s crucial to acknowledge that their reactivity isn't universally advantageous. The very structural feature that contributes to carbocation stability – the presence of three bulky substituents – also introduces a significant constraint: steric hindrance.
Real-World Examples: Tertiary Carbons in Organic Molecules
The theoretical concepts discussed earlier gain significance when viewed through the lens of real-world molecules. Tertiary carbon atoms are not merely abstract entities. They are integral components of numerous organic compounds, influencing their properties and dictating their behavior in chemical reactions.
This section explores specific examples showcasing the diverse roles of tertiary carbons in determining molecular characteristics.
Terpenes: Nature's Building Blocks
Terpenes represent a vast class of natural products synthesized by plants and some insects. Many terpenes feature complex structures built around isoprene units, frequently incorporating tertiary carbon centers.
For example, consider menthol, a cyclic monoterpene responsible for the cooling sensation of mint. The tertiary carbon atoms within menthol's structure contribute to its overall stability. They also play a crucial role in determining its reactivity in various chemical transformations.
The specific arrangement of substituents around these tertiary centers influences menthol's sensory properties and its ability to interact with biological receptors.
Steroids: The Hormonal Messengers
Steroids, another significant class of organic molecules, are characterized by a tetracyclic ring system. These ring systems are abundant with tertiary carbon atoms. Cholesterol, a vital steroid found in animal cell membranes, exemplifies this.
The multiple tertiary carbon atoms within cholesterol's structure contribute to the rigidity of the molecule. This is essential for maintaining cell membrane integrity.
Moreover, the tertiary carbons serve as sites for enzymatic modification. These modifications can alter cholesterol's biological activity or convert it into other steroid hormones like testosterone or cortisol.
The precise stereochemistry at these tertiary centers dictates the specific biological effects of each steroid.
Pharmaceuticals: Drug Design and Tertiary Carbons
Tertiary carbon atoms are frequently incorporated into the structures of pharmaceutical drugs to modulate their properties. These properties include metabolic stability, binding affinity, and overall efficacy.
For instance, atorvastatin (Lipitor), a widely prescribed drug for lowering cholesterol, contains several tertiary carbon atoms.
These centers influence the drug's interaction with the target enzyme, HMG-CoA reductase. This ultimately affects its ability to inhibit cholesterol synthesis.
The strategic placement of tertiary butyl groups or other bulky substituents around a tertiary carbon can also protect the drug molecule from enzymatic degradation. This prolongs its duration of action in the body.
Polymers: Tailoring Material Properties
In polymer chemistry, the presence of tertiary carbon atoms within the polymer backbone or pendant groups can significantly impact the material's physical and chemical properties.
Consider polypropylene, a common thermoplastic polymer. The repeating units contain a tertiary carbon atom.
The presence of this tertiary carbon introduces a degree of branching along the polymer chain. This affects its crystallinity, flexibility, and thermal stability.
By controlling the stereochemistry at the tertiary carbon centers, it is possible to tailor the properties of polypropylene. This makes it suitable for various applications, from packaging materials to fibers.
These examples showcase the diverse and essential roles of tertiary carbon atoms across various fields of chemistry. Their presence influences the properties, reactivity, and biological activity of countless organic molecules.
Understanding the chemistry of tertiary carbons is crucial for designing new molecules with tailored properties. This could be in the development of novel drugs, materials, or catalysts. These real-world applications underscore the fundamental importance of this structural motif in organic chemistry.
Video: Tertiary Carbon: The Hidden Key to Organic Chemistry!
Tertiary Carbon: Frequently Asked Questions
These frequently asked questions will hopefully clarify any confusion about the concept of tertiary carbon.
What exactly is a tertiary carbon?
A tertiary carbon is a carbon atom in an organic molecule that is bonded to three other carbon atoms. This differs from primary (bonded to one other carbon), secondary (bonded to two), and quaternary (bonded to four) carbons. The number of carbon-carbon bonds is what defines a carbon as tertiary.
Why is a tertiary carbon considered "important" in organic chemistry?
The importance stems from its reactivity. The hydrogen atom(s) attached to a tertiary carbon are generally more easily abstracted (removed) during reactions. This is because the resulting tertiary carbocation (if formed) is relatively stable due to the inductive effect of the three alkyl groups bonded to the carbon.
How do I identify a tertiary carbon in a molecule?
Look for a carbon atom directly attached to three other carbon atoms. Count the number of carbon-carbon bonds around each carbon in the molecule. If there are three, you have found a tertiary carbon.
What kind of reactions are tertiary carbons typically involved in?
Tertiary carbons are often involved in SN1 reactions (substitution, nucleophilic, unimolecular) and elimination reactions (E1). Their stability as carbocations during these reaction pathways makes them more likely to participate. The reactivity of tertiary carbon also affects the site of oxidation reactions.