Thermodynamic Favorability: Is It Spontaneous?
The Gibbs Free Energy, a thermodynamic potential that measures the amount of energy available in a thermodynamic system to perform useful work at a constant temperature and pressure, significantly dictates thermodynamic favorability of a reaction. Furthermore, entropy, which is a measure of the disorder or randomness of a system, correlates positively with the spontaneity of reactions, showcasing that systems tend toward maximum entropy given the opportunity. For example, calculations performed using Calorimetry, the science of measuring the heat of chemical reactions or physical changes, can help determine whether a reaction exhibits thermodynamic favorability under specific conditions. Many researchers, such as J. Willard Gibbs, whose contributions to chemical thermodynamics laid the groundwork for our current understanding of free energy, have shown how understanding these principles can help predict if a process will occur spontaneously.
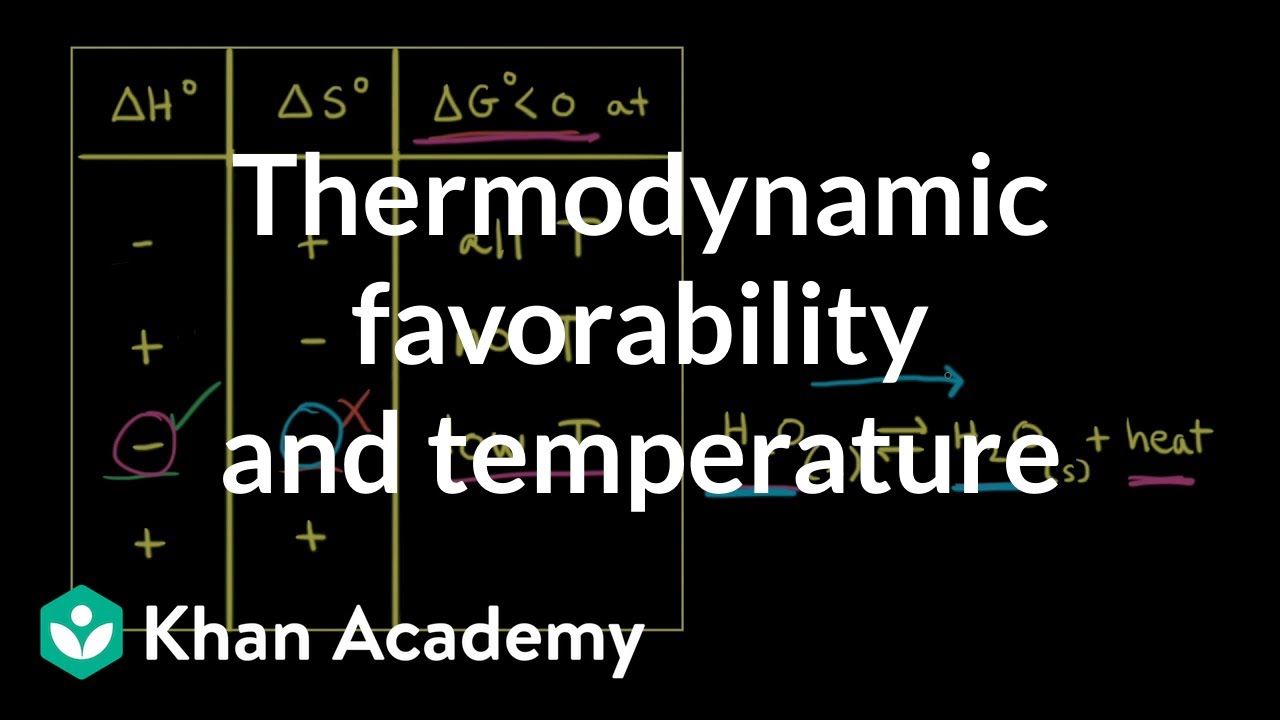
Image taken from the YouTube channel Khan Academy , from the video titled Thermodynamic favorability and temperature | AP Chemistry | Khan Academy .
Thermodynamics: Unveiling the Secrets of Spontaneous Change
Thermodynamics. It's a word that might conjure up images of complex equations or intimidating scientific concepts. But at its heart, thermodynamics is simply the study of energy and how it transforms. Think of it as the science that governs the dance of energy in our universe.
It explores questions like: Why does ice melt at room temperature? Why does a hot cup of coffee eventually cool down? And how can we harness energy to power our world?
What Exactly is Thermodynamics?
Thermodynamics is a broad field, but its core focus is understanding the relationships between heat, work, and energy. It provides a framework for analyzing and predicting the behavior of matter, from the smallest molecules to the largest systems.
It’s built upon a set of fundamental laws that describe how energy is conserved and how it flows. These laws are not just abstract concepts; they have practical applications in countless areas of science and engineering.
The Power of Prediction: Spontaneity
One of the most powerful aspects of thermodynamics is its ability to predict whether a process will occur spontaneously. A spontaneous process is one that happens without any external intervention.
For example, a ball rolling downhill is a spontaneous process. Thermodynamics allows us to determine, under specific conditions, whether a chemical reaction will proceed on its own or whether it requires an input of energy.
This predictive power is crucial in fields like chemistry, materials science, and even biology. Understanding spontaneity enables us to design new materials, optimize chemical reactions, and even gain insights into the processes that drive life itself.
What We'll Explore Together
In this article, we'll embark on a journey to unravel the key concepts of thermodynamics. We'll explore the pioneers who shaped this field and the fundamental principles that govern the behavior of energy.
We will also delve into the concept of thermodynamic potentials, such as Gibbs Free Energy, which provide a powerful tool for predicting spontaneity. By the end, you'll have a solid foundation for understanding the principles of thermodynamics and their far-reaching implications.
The Pioneers of Thermodynamics: Giants on Whose Shoulders We Stand
Before diving into the intricacies of thermodynamic potentials and spontaneity, it's essential to acknowledge the brilliant minds that paved the way. Their groundbreaking work transformed our understanding of energy and laid the foundation for modern thermodynamics. Let's celebrate the pioneers whose insights continue to shape the field.
Sadi Carnot: The Visionary of Heat Engines
Nicolas Léonard Sadi Carnot (1796-1832), often hailed as the “father of thermodynamics,” possessed an almost clairvoyant understanding of heat engines.
His Reflections on the Motive Power of Fire (1824) presented the concept of an ideal heat engine, now known as the Carnot engine.
Carnot's engine operates on a reversible cycle, achieving maximum efficiency between two heat reservoirs.
Although he predated the formal establishment of the First Law of Thermodynamics, his work foreshadowed its importance.
His contribution was revolutionary because it underscored the limits of converting heat into work and set the stage for understanding the fundamental laws governing energy transformations.
Rudolf Clausius: Unveiling Entropy
Rudolf Clausius (1822-1888) was instrumental in formulating the Second Law of Thermodynamics.
He introduced the concept of entropy, denoted by the symbol S, as a measure of the disorder or randomness of a system.
Clausius famously stated that “the entropy of the universe tends to a maximum.”
This insightful assertion implies that spontaneous processes increase the overall disorder in the universe.
His mathematical treatment of entropy provided a quantitative way to assess the spontaneity of processes.
Clausius bridged the gap between abstract theory and practical applications.
Ludwig Boltzmann: Bridging the Microscopic and Macroscopic
Ludwig Boltzmann (1844-1906) took a revolutionary step by connecting thermodynamics with statistical mechanics.
He formulated the relationship between entropy (S) and the number of microstates (Ω) corresponding to a given macrostate: S = kB ln Ω.
Here, kB represents the Boltzmann constant.
This equation beautifully illustrates that entropy is directly proportional to the number of possible microscopic arrangements that can result in the same macroscopic state.
Boltzmann's statistical approach provided a deeper understanding of entropy as a measure of probability and disorder at the molecular level.
This conceptual leap bridged the gap between the microscopic world of atoms and molecules and the macroscopic world of observable thermodynamic properties.
Hermann von Helmholtz: Defining Free Energy
Hermann von Helmholtz (1821-1894) made significant contributions to numerous scientific fields, including thermodynamics.
He introduced the concept of Helmholtz Free Energy (A), defined as A = U - TS, where U is the internal energy, T is the absolute temperature, and S is the entropy of the system.
Helmholtz Free Energy is particularly useful for determining the spontaneity of processes occurring at constant temperature and volume.
A decrease in Helmholtz Free Energy indicates that a process is spontaneous under these conditions.
Helmholtz’s work provided a powerful tool for analyzing and predicting the behavior of systems in various physical and chemical transformations.
Willard Gibbs: The Architect of Chemical Thermodynamics
Josiah Willard Gibbs (1839-1903) is widely regarded as the founder of chemical thermodynamics.
He unified the principles of thermodynamics with chemical phenomena.
Gibbs introduced the concept of Gibbs Free Energy (G), defined as G = H - TS, where H is the enthalpy, T is the absolute temperature, and S is the entropy of the system.
Gibbs Free Energy is the cornerstone for determining the spontaneity of processes at constant temperature and pressure.
A decrease in Gibbs Free Energy indicates that a process is spontaneous under these conditions.
Gibbs' work provided a comprehensive framework for understanding chemical equilibria, phase transitions, and the behavior of mixtures, fundamentally shaping the field of chemistry.
Continuing the Legacy: Modern Researchers
While these pioneers laid the groundwork, the field of thermodynamics continues to evolve thanks to the work of modern researchers. Their contributions expand our understanding of complex systems and enable technological advancements.
Their groundbreaking work pushes the boundaries of thermodynamics, ensuring that this vital field remains dynamic and relevant in addressing the challenges of the 21st century.
Fundamental Concepts in Thermodynamics
Before we can wield the powerful tools of thermodynamic potentials to predict spontaneity, we must first firmly grasp the foundational concepts upon which thermodynamics is built. Think of these as the essential building blocks; without them, our understanding will be incomplete. So, let's dive in and explore these core ideas together!
Thermodynamics: The Science of Energy Transformation
At its heart, thermodynamics is the study of energy and its transformations. It provides us with a framework to understand how energy flows, how it can be converted from one form to another, and the limitations imposed by the laws of nature on these processes.
It's a powerful tool for predicting whether a process will occur spontaneously and for determining the conditions under which a reaction will reach equilibrium. Thermodynamics is governed by a few fundamental laws:
- The First Law states that energy is conserved.
- The Second Law introduces the concept of entropy and dictates that spontaneous processes increase the total entropy of the universe.
- The Third Law defines the absolute zero of entropy.
Understanding these laws is crucial for comprehending any thermodynamic system.
Spontaneity: Will It Happen on Its Own?
Spontaneity refers to the inherent tendency of a process to occur without needing continuous external influence. A spontaneous process will proceed on its own, given the right conditions.
It is important to note that spontaneity does not mean instantaneous. A spontaneous reaction can still be very slow. Rusting of iron is a classic example of a spontaneous yet slow process.
Several factors influence spontaneity:
- Enthalpy (ΔH): Exothermic reactions (ΔH < 0) often favor spontaneity, as they release energy.
- Entropy (ΔS): An increase in entropy (ΔS > 0) also favors spontaneity, as it signifies increased disorder.
- Temperature (T): Temperature plays a crucial role, especially when both enthalpy and entropy changes are significant.
Entropy Change of the Universe (ΔSuniverse): The Ultimate Criterion
The Second Law of Thermodynamics provides the ultimate yardstick for determining spontaneity: the entropy change of the universe (ΔSuniverse).
For a process to be spontaneous, the total entropy of the universe must increase. Mathematically, this is expressed as:
ΔSuniverse = ΔSsystem + ΔSsurroundings > 0
If ΔSuniverse is positive, the process is spontaneous. If it's negative, the process is non-spontaneous. And if it's zero, the system is at equilibrium.
Defining the System and Surroundings
In thermodynamics, we always focus on a specific part of the universe that we are interested in studying: the system. The system is where the process or reaction of interest is taking place.
Everything else outside the system is considered the surroundings. The system and surroundings together constitute the universe.
Defining the system and surroundings clearly is vital for proper analysis. The exchange of energy and matter between the system and surroundings determines the type of system (open, closed, or isolated).
State Functions: Path Independence
State functions are properties that depend only on the initial and final states of the system, not on the path taken to reach those states.
Think of it like hiking a mountain: The change in altitude (a state function) only depends on your starting and ending points, not the specific trail you took.
Examples of state functions include:
- Internal energy (U)
- Enthalpy (H)
- Entropy (S)
- Gibbs Free Energy (G)
The path independence of state functions simplifies thermodynamic calculations considerably.
Thermodynamic Potentials: Unveiling Equilibrium
Thermodynamic potentials are state functions that provide valuable information about the equilibrium conditions of a system. They essentially tell us the maximum amount of work that can be extracted from a system under certain constraints.
The most commonly used thermodynamic potentials are:
- Internal energy (U)
- Enthalpy (H)
- Helmholtz Free Energy (A)
- Gibbs Free Energy (G)
Each potential is most useful under specific conditions (constant volume, constant pressure, etc.) for determining whether a process is spontaneous or at equilibrium.
Chemical Potential: Compositional Changes
The chemical potential (μ) is a crucial concept when dealing with systems of varying composition. It represents the change in Gibbs Free Energy (G) of a system with respect to the change in the amount of a particular component, while keeping temperature, pressure, and the amounts of other components constant.
Mathematically:
μi = (∂G/∂ni)T,P,nj≠i
Where:
- μi is the chemical potential of component i
- G is the Gibbs Free Energy
- ni is the amount (moles) of component i
- T is temperature
- P is pressure
- nj≠i represents the amounts of all other components (j) in the system
The chemical potential is essential for understanding phase equilibria, chemical reactions, and other processes involving changes in composition. It essentially dictates the direction in which a system will evolve to minimize its Gibbs Free Energy.
Thermodynamic Potentials: The Keys to Predicting Spontaneity
Before we can wield the powerful tools of thermodynamic potentials to predict spontaneity, we must first firmly grasp the foundational concepts upon which thermodynamics is built. Think of these as the essential building blocks; without them, our understanding will be incomplete. So, let's dive in and explore…
Thermodynamic potentials are state functions that provide a measure of the energy available in a system to do useful work. Understanding these potentials is crucial for predicting whether a process will occur spontaneously under specific conditions.
Let's explore the two most prominent thermodynamic potentials, Gibbs Free Energy and Helmholtz Free Energy, and how they act as our keys to unlocking the secrets of spontaneity.
Gibbs Free Energy (ΔG): Spontaneity at Constant Temperature and Pressure
Gibbs Free Energy (G) is perhaps the most widely used thermodynamic potential, particularly in chemistry and biology. It elegantly combines enthalpy, entropy, and temperature into a single value that predicts spontaneity at constant temperature and pressure.
The change in Gibbs Free Energy (ΔG) during a process directly indicates whether that process is spontaneous:
-
If ΔG < 0: The process is spontaneous (or thermodynamically favorable) in the forward direction.
-
If ΔG > 0: The process is non-spontaneous in the forward direction, but spontaneous in the reverse direction.
-
If ΔG = 0: The system is at equilibrium, with no net change occurring.
The Gibbs Free Energy Equation: ΔG = ΔH - TΔS
The Gibbs Free Energy is defined by the following equation:
ΔG = ΔH - TΔS
Where:
- ΔG is the change in Gibbs Free Energy
- ΔH is the change in Enthalpy
- T is the absolute temperature (in Kelvin)
- ΔS is the change in Entropy
This equation beautifully illustrates how enthalpy, entropy, and temperature interplay to determine the spontaneity of a process.
Enthalpy (ΔH): The Heat Content
Enthalpy (H) represents the heat content of a system at constant pressure. A negative ΔH indicates an exothermic process, where heat is released to the surroundings, making the process more favorable.
Conversely, a positive ΔH indicates an endothermic process, where heat is absorbed from the surroundings, making the process less favorable.
Entropy (ΔS): The Measure of Disorder
Entropy (S) is a measure of the disorder or randomness of a system. The Second Law of Thermodynamics dictates that the entropy of the universe always increases for a spontaneous process.
-
A positive ΔS indicates an increase in disorder, which generally favors spontaneity.
-
A negative ΔS indicates a decrease in disorder, which generally opposes spontaneity.
Helmholtz Free Energy (ΔA): Spontaneity at Constant Temperature and Volume
While Gibbs Free Energy reigns supreme at constant temperature and pressure, the Helmholtz Free Energy (A) plays a similar role when volume is held constant.
The change in Helmholtz Free Energy (ΔA) predicts spontaneity under these conditions:
-
If ΔA < 0: The process is spontaneous at constant temperature and volume.
-
If ΔA > 0: The process is non-spontaneous at constant temperature and volume.
-
If ΔA = 0: The system is at equilibrium.
The Helmholtz Free Energy is particularly useful in situations involving closed systems with fixed volumes, such as combustion reactions in a sealed container. While less frequently used than Gibbs Free Energy in everyday chemistry, it remains a vital tool for specific applications.
Equilibrium: The Balancing Act
Thermodynamic Potentials: The Keys to Predicting Spontaneity
Before we can wield the powerful tools of thermodynamic potentials to predict spontaneity, we must first firmly grasp the foundational concepts upon which thermodynamics is built. Think of these as the essential building blocks; without them, our understanding will be incomplete. So, let's delve into the fascinating world of equilibrium, where opposing forces dance in perfect harmony.
Defining Equilibrium: Where ΔG = 0
In the grand theater of thermodynamics, equilibrium isn't merely a static state. It is a dynamic state.
Imagine a seesaw perfectly balanced: even though it appears motionless, subtle adjustments are constantly being made to maintain that balance.
Similarly, in a system at equilibrium, the rates of the forward and reverse processes are equal.
This means that there's no net change in the system's properties over time. Reactions continue to occur, but at equal and opposite rates.
A crucial indicator of equilibrium is the Gibbs Free Energy (ΔG).
At equilibrium, ΔG = 0. This signifies that the system is at its lowest possible energy state. No further spontaneous change can occur under the given conditions.
Factors Influencing Equilibrium: A Delicate Dance
Equilibrium isn't set in stone; it's a delicate balance that can be influenced by external factors. Changes in temperature, pressure, and concentration can shift the equilibrium position, favoring either the forward or reverse reaction.
Let's explore these factors:
Temperature: Shifting the Balance
Temperature plays a crucial role in influencing equilibrium.
According to Le Chatelier's Principle, increasing the temperature will favor the reaction that absorbs heat (endothermic reaction).
Conversely, decreasing the temperature will favor the reaction that releases heat (exothermic reaction).
Think of it as the system trying to counteract the change you've imposed upon it. If you add heat, it will try to use that heat up; if you remove heat, it will try to generate more.
Pressure: Compressing the Possibilities
Pressure primarily affects equilibrium in systems involving gases.
Increasing the pressure will favor the side of the reaction with fewer moles of gas.
Conversely, decreasing the pressure will favor the side with more moles of gas.
Imagine squeezing a balloon; the system will try to reduce the volume to alleviate the pressure.
Concentration: The Push and Pull of Reactants and Products
Changing the concentration of reactants or products will also shift the equilibrium.
Increasing the concentration of reactants will favor the forward reaction, pushing the equilibrium towards product formation.
Increasing the concentration of products will favor the reverse reaction, pushing the equilibrium back towards reactant formation.
It's like adding more weight to one side of a scale; the balance will shift in the opposite direction.
Video: Thermodynamic Favorability: Is It Spontaneous?
FAQs: Thermodynamic Favorability: Is It Spontaneous?
What does "thermodynamically favorable" mean?
Thermodynamically favorable means a reaction or process will release free energy (negative ΔG) and move towards equilibrium. It indicates a potential for change under given conditions.
Does thermodynamically favorable always mean spontaneous?
Not necessarily. Thermodynamic favorability indicates a tendency to occur. Spontaneity refers to whether a reaction actually proceeds at a noticeable rate. Kinetics play a crucial role; a large activation energy can prevent a thermodynamically favorable reaction from occurring quickly.
What factors influence thermodynamic favorability?
Thermodynamic favorability is primarily determined by changes in enthalpy (ΔH) and entropy (ΔS), as reflected in the Gibbs Free Energy equation (ΔG = ΔH - TΔS). Temperature (T) is also a major factor. A negative ΔG indicates thermodynamic favorability.
If a reaction isn't spontaneous, can it still be thermodynamically favorable?
Yes, a reaction can be thermodynamically favorable but not spontaneous if it has a high activation energy. Even though the reaction releases energy overall (negative ΔG, indicating thermodynamic favorability), the energy barrier prevents it from proceeding at an observable rate without external energy input.
So, the next time you're wondering if a reaction will actually happen just because it's thermodynamically favorable, remember there's more to the story than just spontaneity. Understanding the difference between thermodynamic favorability and kinetics can save you a lot of time and frustration in the lab (or even in the kitchen!). Keep experimenting, and keep asking questions!