Zone of Saturation: Upper Boundary Guide
The water table, a fluctuating surface, represents the upper boundary of the zone of saturation, an area crucial for understanding groundwater resources and is often mapped using data from organizations like the US Geological Survey (USGS). Hydraulic conductivity, a key parameter, influences the rate at which groundwater flows within this saturated zone, impacting the position of the water table, which can be modeled using tools like MODFLOW, a widely-used groundwater simulation program. The work of Henry Darcy laid the foundation for understanding groundwater flow, which is essential for characterizing the zone of saturation and defining its upper limit in various geological formations.
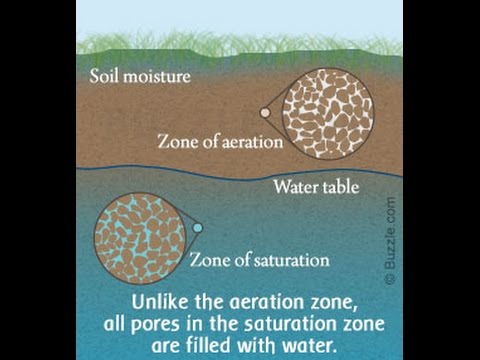
Image taken from the YouTube channel Get Science & Technology , from the video titled Difference Between Zone of Aeration and Zone of Saturation .
The Hidden World of Groundwater: A Vital Resource Under Pressure
Groundwater, often unseen and underestimated, is an indispensable component of the Earth's intricate water cycle. This subterranean reservoir, residing within the pores and fractures of rocks and sediments, plays a pivotal role in sustaining life and supporting human activities.
Groundwater's Role in the Water Cycle
Unlike readily visible surface water sources, groundwater exists as a hidden reserve, constantly interacting with other parts of the water cycle.
It is recharged by precipitation that infiltrates the soil and percolates downwards, gradually replenishing aquifers. Simultaneously, groundwater discharges into surface water bodies like rivers, lakes, and wetlands, maintaining their baseflow and ecological health, especially during dry periods.
This dynamic interplay underscores the interconnectedness of surface and subsurface water resources, highlighting the need for a holistic approach to water management.
The Importance of Groundwater: A Lifeline for Humanity
Groundwater's significance extends far beyond its role in the natural environment. It serves as a primary source of freshwater for a multitude of human uses.
-
Drinking Water: Millions of people rely on groundwater for their daily water needs, particularly in rural areas and regions with limited surface water availability. Its natural filtration through geological formations often results in higher water quality compared to surface sources, reducing the need for extensive treatment.
-
Agriculture: Groundwater irrigation is crucial for crop production, especially in arid and semi-arid regions. It provides a reliable water supply during droughts, ensuring food security and supporting agricultural economies.
-
Industry: Many industries depend on groundwater for various processes, including manufacturing, cooling, and cleaning. Its consistent temperature and chemical composition make it a valuable resource for industrial applications.
The Imperative of Responsible Groundwater Management
The increasing demand for water, coupled with the threat of contamination, necessitates a paradigm shift towards responsible groundwater management.
Over-extraction of groundwater can lead to a range of detrimental consequences, including:
-
Water Table Depletion: Excessive pumping can lower the water table, increasing pumping costs and potentially drying up wells.
-
Land Subsidence: In some areas, groundwater depletion can cause the land surface to sink, damaging infrastructure and increasing flood risk.
-
Saltwater Intrusion: In coastal regions, over-pumping can draw saltwater into freshwater aquifers, rendering them unusable for drinking and irrigation.
Furthermore, groundwater is vulnerable to contamination from various sources, including:
-
Agricultural Runoff: Fertilizers and pesticides can leach into groundwater, polluting aquifers and posing risks to human health.
-
Industrial Discharges: Improper disposal of industrial waste can contaminate groundwater with toxic chemicals.
-
Leaky Underground Storage Tanks: Gasoline and other fuels can leak from underground tanks, contaminating groundwater and creating environmental hazards.
Effective groundwater management requires a comprehensive approach that includes:
-
Monitoring and Assessment: Regularly monitoring groundwater levels and quality to track changes and identify potential problems.
-
Regulation and Enforcement: Implementing regulations to control groundwater extraction and prevent contamination.
-
Public Awareness and Education: Educating the public about the importance of groundwater and the need for responsible water use.
Only through a concerted effort can we ensure the sustainable use of this precious resource for generations to come.
Key Components: Unveiling the Anatomy of a Groundwater System
Understanding the intricate workings of a groundwater system requires a deep dive into its essential building blocks. Just as a body comprises organs and tissues, a groundwater system consists of distinct zones and formations that dictate how water is stored, flows, and interacts with the surrounding environment. Let's explore these key components to gain a comprehensive understanding of the anatomy of groundwater.
The Water Table: Defining the Upper Boundary
The water table represents the upper surface of the saturated zone, the area where all pore spaces within the soil or rock are completely filled with water. Think of it as the dividing line between the unsaturated zone above and the fully saturated zone below.
This boundary is not static; it's a dynamic interface that reflects the balance between groundwater recharge and discharge. Its depth and shape significantly influence groundwater availability and flow patterns.
Factors Influencing Water Table Depth
The depth of the water table is far from constant; it varies considerably depending on a number of interconnected factors.
Rainfall patterns play a crucial role; periods of heavy precipitation typically lead to a rise in the water table as more water infiltrates the ground.
Geology is also a key determinant. Permeable soils and rocks allow water to percolate more easily, potentially leading to a shallower water table.
Conversely, impermeable layers can impede infiltration and result in a deeper water table. Land use practices, such as deforestation or urbanization, can also alter infiltration rates and affect water table depths.
Water Table Fluctuations: A Dynamic Equilibrium
The water table is not a static line; it's constantly fluctuating in response to changes in recharge and discharge rates. During periods of heavy rainfall or snowmelt, the water table typically rises as more water replenishes the groundwater reservoir.
Conversely, during prolonged dry periods or increased groundwater extraction, the water table tends to decline. These fluctuations can have significant implications for water availability and ecosystem health. Understanding these dynamics is crucial for sustainable groundwater management.
Zone of Aeration (Vadose Zone): The Unsaturated Realm
Above the water table lies the zone of aeration, also known as the vadose zone. This is the unsaturated zone where the pore spaces are only partially filled with water, with the remaining space occupied by air.
The vadose zone plays a critical role in the infiltration and percolation of water from the surface down to the saturated zone.
Water Movement Through the Vadose Zone
As water infiltrates from the surface, it moves through the vadose zone via gravity and capillary action. The rate of movement depends on factors such as soil type, vegetation cover, and the amount of water already present in the soil.
Types of Water in the Vadose Zone
Several types of water are found in the vadose zone. Soil moisture is held tightly to soil particles by surface tension. Gravity water is the water that moves downwards due to gravity.
These different forms of water play distinct roles in supporting plant life and replenishing groundwater resources.
Capillary Fringe: Bridging the Gap
Directly above the water table, a zone known as the capillary fringe exists. This is a narrow band where water is drawn upwards from the saturated zone by capillary action, a phenomenon caused by the surface tension of water molecules.
Capillary Action: defying Gravity
Capillary action allows water to move upwards against the force of gravity, filling the pore spaces in the soil or rock above the water table. The height of the capillary fringe depends on the pore size of the material; finer-grained materials tend to have a higher capillary fringe than coarser-grained materials.
Relationship to the Water Table and Vadose Zone
The capillary fringe forms a transitional zone between the fully saturated conditions of the water table and the unsaturated conditions of the vadose zone. It plays a crucial role in connecting these two zones and influencing the movement of water and solutes between them.
Aquifers: The Underground Reservoirs
Aquifers are saturated geological formations that are permeable enough to store and transmit significant quantities of groundwater. They are the primary sources of groundwater for human use.
Porosity and Permeability: Key Characteristics
Two key characteristics define an aquifer's ability to store and transmit water: porosity and permeability. Porosity refers to the amount of empty space (pores or fractures) within the rock or sediment.
Permeability refers to the interconnectedness of these spaces, which determines how easily water can flow through the material. High porosity and permeability are essential for an aquifer to be productive.
Confined vs. Unconfined Aquifers
Aquifers can be classified as either confined or unconfined, depending on the presence or absence of a confining layer (an impermeable layer of rock or sediment). Unconfined aquifers, also known as water table aquifers, are directly connected to the surface and are recharged by rainfall and infiltration.
Confined aquifers, on the other hand, are bounded above and below by confining layers. They are typically recharged at a distance from the confined area and may be under pressure.
Hydraulic Properties: Transmissivity and Storativity
Transmissivity is a measure of how much water can flow horizontally through an aquifer. Storativity is a measure of the amount of water an aquifer releases from or takes into storage per unit surface area per unit change in hydraulic head. These properties are essential for assessing the potential yield of an aquifer and for managing groundwater resources sustainably.
Groundwater Dynamics: Recharge and Discharge
Understanding groundwater systems requires understanding how water enters (recharge) and exits (discharge) aquifers. These dynamic processes dictate the overall health and sustainability of this vital resource.
A balanced system ensures long-term availability, while imbalances can lead to depletion or other environmental issues.
Groundwater Recharge: Replenishing the Source
Groundwater recharge is the process by which water infiltrates the earth's surface and replenishes aquifers. It's the primary mechanism for sustaining groundwater resources.
Without adequate recharge, aquifers would gradually deplete, impacting water availability for various uses.
Sources of Recharge
Several sources contribute to groundwater recharge, each playing a significant role depending on the local environment.
Rainfall is perhaps the most obvious and widespread source, with rainwater percolating through the soil and into underlying aquifers. Snowmelt can be a substantial contributor in colder climates, as the melting snow slowly releases water that infiltrates the ground. Stream infiltration occurs when surface water from rivers and streams seeps into adjacent aquifers, especially where streambeds are permeable.
Factors Affecting Recharge Rates
The rate at which recharge occurs is influenced by a complex interplay of factors.
Soil type plays a crucial role, with sandy soils allowing for faster infiltration than clay-rich soils. Vegetation cover can both enhance and impede recharge, as plant roots can create pathways for water to enter the soil, while dense vegetation can also intercept rainfall and reduce the amount reaching the ground. Land use practices have a significant impact, with urban development often reducing recharge due to impervious surfaces like roads and buildings.
Artificial Recharge
In areas where natural recharge is insufficient, artificial recharge techniques can be employed to supplement groundwater supplies.
These methods involve intentionally diverting surface water or treated wastewater into the ground to replenish aquifers. Examples include infiltration basins, injection wells, and rainwater harvesting systems. Artificial recharge can be a valuable tool for managing water resources in water-stressed regions.
Groundwater Discharge: Exiting the Aquifer
Groundwater discharge refers to the ways in which groundwater exits an aquifer. This can occur naturally or through human intervention.
Understanding discharge mechanisms is crucial for managing groundwater sustainably.
Natural Discharge Points
Several natural features serve as discharge points for groundwater.
Springs occur where groundwater emerges at the surface, often forming pools or streams. Seeps are similar to springs but involve a more diffuse discharge of groundwater over a larger area. Baseflow to streams is the contribution of groundwater to the flow of rivers and streams, helping to maintain streamflow even during dry periods.
Groundwater Extraction by Humans
Humans extract groundwater through wells for a variety of purposes.
Wells provide access to groundwater for drinking water, irrigation, and industrial uses. Over-extraction of groundwater can lead to depletion of aquifers, land subsidence, and saltwater intrusion in coastal areas.
Evapotranspiration
Evapotranspiration is another discharge mechanism. It involves the combined processes of evaporation from the soil surface and transpiration from plants. In areas with shallow water tables, evapotranspiration can be a significant factor in groundwater discharge.
Measuring the Invisible: Techniques for Groundwater Monitoring
Understanding groundwater systems requires understanding how water enters (recharge) and exits (discharge) aquifers. These dynamic processes dictate the overall health and sustainability of this vital resource.
A balanced system ensures long-term availability, while imbalances can lead to depletion or other environmental consequences. But how do scientists and engineers actually see what's happening beneath the surface? Groundwater monitoring relies on a suite of specialized techniques to measure its characteristics and track its behavior.
Piezometers: Sentinels of Groundwater Pressure
Piezometers are simple yet crucial tools for measuring groundwater pressure, also known as hydraulic head.
These devices, typically small-diameter pipes with a screened interval at the bottom, are installed in the ground to allow water to enter.
The water level inside the piezometer represents the hydraulic head at that location, providing a direct measure of the potential energy of the groundwater.
Determining Groundwater Flow Directions
By installing a network of piezometers in an area, scientists can determine the direction of groundwater flow.
Water flows from areas of high hydraulic head to areas of low hydraulic head, and piezometer measurements provide the data needed to map these flow paths.
This information is vital for understanding how contaminants might spread or how groundwater resources are replenished.
Piezometer Design and Installation
The design and installation of piezometers are critical to ensuring accurate measurements.
The screened interval must be properly sized to allow water to enter freely while preventing sediment from clogging the device.
The piezometer must also be carefully sealed to prevent surface water from infiltrating and skewing the readings.
Proper development after installation ensures the piezometer is hydraulically connected to the surrounding aquifer.
Monitoring Wells: Guardians of Groundwater Quality
Monitoring wells serve a dual purpose: tracking groundwater levels and assessing water quality.
While similar in construction to piezometers, monitoring wells are typically designed with larger diameters to facilitate water sample collection.
These wells are strategically placed to provide a representative picture of groundwater conditions in a particular area.
Detecting and Assessing Groundwater Contamination
One of the most important uses of monitoring wells is to detect and assess groundwater contamination.
By regularly collecting water samples and analyzing them for various pollutants, scientists can identify sources of contamination, track the movement of contaminant plumes, and evaluate the effectiveness of remediation efforts.
Early detection is crucial for minimizing the impact of contamination on human health and the environment.
Design and Placement Considerations
The design and placement of monitoring wells are critical for obtaining reliable data.
Wells should be constructed with materials that do not leach contaminants into the water sample.
The well screen should be positioned to intercept the zone of interest, and the well should be properly developed to remove any drilling fluids or sediment.
The placement of wells should be based on a thorough understanding of the hydrogeology of the area, with wells strategically located to monitor potential sources of contamination and to track the movement of groundwater.
Water Level Recorders (Pressure Transducers): Continuous Monitoring
Water level recorders, often using pressure transducers, offer a significant advantage over manual measurements: continuous monitoring of groundwater levels.
These devices are installed in wells or piezometers and automatically record water levels at predetermined intervals.
This allows for a much more detailed understanding of groundwater fluctuations over time.
Automated Water Level Measurement
Pressure transducers measure the pressure exerted by the column of water above them.
This pressure is then converted to a water level reading, which is stored in the device's memory.
Data loggers allow for long-term, unattended monitoring.
Data Collection and Analysis
The data collected by water level recorders can be downloaded and analyzed to identify trends, assess the impact of pumping or recharge events, and calibrate groundwater models.
Continuous monitoring provides a more complete picture of groundwater dynamics than periodic manual measurements, leading to better informed management decisions.
Careful data analysis, including quality control and correction for barometric pressure changes, is essential for accurate interpretation of water level data.
Key Groundwater Property: Hydraulic Conductivity
Understanding groundwater systems requires understanding how water enters (recharge) and exits (discharge) aquifers. These dynamic processes dictate the overall health and sustainability of this vital resource.
A balanced system ensures long-term availability, while imbalances can lead to depletion or contamination. However, recharge and discharge are not the only critical elements. At the heart of any groundwater analysis lies the concept of hydraulic conductivity, a property that dictates the rate at which groundwater moves.
What is Hydraulic Conductivity?
Hydraulic conductivity (K) is a quantitative measure of a geological material's ability to transmit water. It represents the volume of water that will flow through a unit area of an aquifer under a unit hydraulic gradient. In simpler terms, it tells us how easily water can flow through soil or rock.
A high hydraulic conductivity means water can move rapidly, while a low value indicates slow movement. This property is fundamental to understanding groundwater flow patterns, predicting contaminant transport, and managing groundwater resources effectively.
Units of Hydraulic Conductivity
Hydraulic conductivity is typically expressed in units of length per time. The most common units are meters per day (m/day) or feet per day (ft/day). Other units, such as centimeters per second (cm/s), may be used in specific contexts.
It is important to pay close attention to the units when comparing hydraulic conductivity values or performing calculations. Inconsistencies in units can lead to significant errors in groundwater assessments.
Factors Influencing Hydraulic Conductivity
Several factors influence the hydraulic conductivity of a geological material. Understanding these factors is crucial for accurately characterizing groundwater systems.
Grain Size and Sorting
The size and sorting of particles within a soil or rock formation are major determinants of hydraulic conductivity. Larger particles generally create larger pore spaces, allowing for easier water flow.
Well-sorted materials, where particles are of similar size, tend to have higher hydraulic conductivity than poorly sorted materials with a mixture of particle sizes. Poor sorting can result in smaller particles filling the gaps between larger particles, reducing pore space and impeding water flow.
Fracture Density
In fractured rock formations, the density and interconnectedness of fractures significantly influence hydraulic conductivity. Fractures act as conduits for groundwater flow, and a higher fracture density typically leads to higher hydraulic conductivity.
The orientation and aperture (width) of fractures also play a role. Wide, interconnected fractures oriented in the direction of groundwater flow will contribute to higher hydraulic conductivity than narrow, isolated fractures.
Other Key Influences
- Porosity: The total volume of pore space in a material.
- Fluid Viscosity: Higher viscosity fluids flow less readily.
- Degree of Saturation: Unsaturated conditions reduce conductivity.
Determining Hydraulic Conductivity: Common Methods
Several methods are available for determining hydraulic conductivity in the field and laboratory. The choice of method depends on the scale of investigation, the type of geological material, and the available resources.
Slug Tests
Slug tests are relatively simple and inexpensive field methods used to estimate hydraulic conductivity in individual wells. A known volume of water (a "slug") is rapidly added to or removed from a well, and the subsequent water level change is monitored over time.
The rate at which the water level recovers to its static level is used to estimate hydraulic conductivity near the well screen. Slug tests are particularly useful for characterizing the hydraulic properties of shallow, unconfined aquifers.
Pumping Tests
Pumping tests involve pumping water from a well at a constant rate and monitoring the drawdown (water level decline) in the pumping well and in nearby observation wells. Analyzing the drawdown data allows for the estimation of hydraulic conductivity and other aquifer parameters, such as storativity.
Pumping tests provide a more integrated measure of hydraulic conductivity over a larger area than slug tests. They are commonly used to characterize the hydraulic properties of both confined and unconfined aquifers.
Visualizing the Water Table: Creating and Using Water Table Maps
Understanding groundwater systems requires understanding how water enters (recharge) and exits (discharge) aquifers. These dynamic processes dictate the overall health and sustainability of this vital resource.
A balanced system ensures long-term availability, while imbalances can lead to depletion or contamination. To effectively manage this hidden resource, scientists and engineers rely on various tools, one of the most insightful being the water table map. This section explores the creation and application of these maps, illuminating their crucial role in groundwater management and resource assessment.
What are Water Table Maps?
Water table maps are essentially contour maps that depict the elevation of the water table across a specific area. They provide a visual representation of the groundwater surface, allowing users to understand its three-dimensional form and slope.
These maps are constructed using groundwater level measurements obtained from wells and piezometers strategically placed across the region of interest. The accuracy and detail of the map depend heavily on the density and distribution of these monitoring points.
Creating a Water Table Map: Contouring Groundwater Levels
The creation of a water table map involves several key steps:
- Data Collection: First, accurate groundwater level measurements must be obtained from wells and piezometers. These measurements represent the elevation of the water table at specific locations.
- Data Processing: The collected data are then processed and organized. This may involve correcting for any discrepancies or inconsistencies in the measurements.
- Contouring: The heart of the process lies in contouring. Contours are lines that connect points of equal groundwater elevation. By drawing these lines, a visual representation of the water table surface emerges.
- Map Production: Finally, the contours are overlaid on a base map that includes geographic features, such as rivers, roads, and topography. This provides context and enhances the map's interpretability.
Various software packages and techniques are available for creating water table maps, ranging from manual drafting to sophisticated geostatistical modeling. The choice of method depends on the desired level of accuracy and the available data.
Applications in Groundwater Management and Resource Assessment
Water table maps are invaluable tools for a wide range of applications in groundwater management and resource assessment. They allow professionals to:
- Understand flow dynamics.
- Make informed decisions.
- Protect this precious resource.
Identifying Recharge and Discharge Areas
One of the primary uses of water table maps is to identify areas of groundwater recharge and discharge. Recharge areas are locations where water infiltrates the ground and replenishes the aquifer. These areas are typically characterized by higher water table elevations.
Discharge areas, on the other hand, are locations where groundwater flows out of the aquifer, such as springs, streams, or wetlands. These areas typically exhibit lower water table elevations. By analyzing the contours on a water table map, scientists can pinpoint these critical zones.
Understanding Groundwater Flow Directions
Water table maps also provide insights into groundwater flow directions. Groundwater flows from areas of higher hydraulic head (higher water table elevation) to areas of lower hydraulic head (lower water table elevation).
The direction of groundwater flow is always perpendicular to the contour lines, pointing downhill. By examining the contour patterns, one can trace the pathways that groundwater takes as it moves through the subsurface. This information is crucial for predicting the movement of contaminants and managing groundwater resources effectively.
In conclusion, water table maps serve as a powerful visualization tool to aid in understanding, managing, and protecting groundwater resources.
Video: Zone of Saturation: Upper Boundary Guide
Frequently Asked Questions: Zone of Saturation - Upper Boundary Guide
What exactly does "zone of saturation" mean?
The zone of saturation is the area underground where all pore spaces in the soil and rock are completely filled with water. It's the area that feeds wells and springs. The water table marks the upper boundary of the zone of saturation.
How is the top of the saturated zone determined?
The upper boundary of the zone of saturation is defined by the water table. This is the level at which the water pressure in the ground equals atmospheric pressure. Well water levels accurately indicate the water table's location.
Why is it important to understand the water table?
Understanding the water table, or the upper boundary of the zone of saturation, is crucial for managing groundwater resources. It helps in activities like well placement, predicting flooding, and assessing the potential for groundwater contamination.
Can the upper boundary of the zone of saturation change?
Yes, the water table, defining the upper boundary of the zone of saturation, fluctuates. Rainfall, drought, pumping rates from wells, and other factors cause it to rise and fall over time. Monitoring these changes is important for responsible water resource management.
So, there you have it! Understanding the upper boundary of the zone of saturation is key to responsible water management and infrastructure planning. Hopefully, this guide has given you a clearer picture – now go forth and hydrate responsibly!