What Enzyme Breaks Down Starch? Amylase Guide
The human digestive system relies on a class of proteins known as enzymes. Amylase, an enzyme produced primarily in the salivary glands and pancreas, facilitates the breakdown of starch into simpler sugars. Starch digestion, a vital part of human nutrition, begins in the mouth, where salivary amylase initiates the hydrolysis of alpha 1-4 glycosidic bonds in starch molecules. Understanding what enzyme breaks down starch involves exploring the functions of both salivary and pancreatic amylase, as well as related enzymes, to effectively convert complex carbohydrates into glucose.
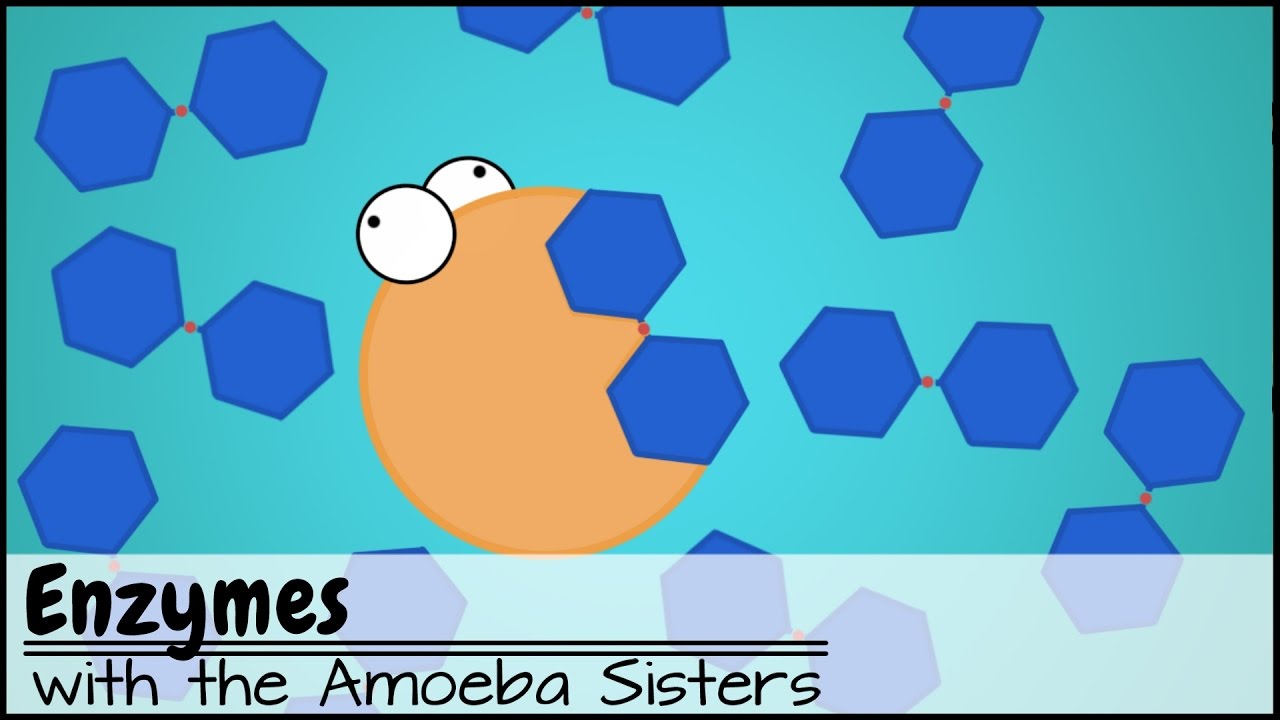
Image taken from the YouTube channel Amoeba Sisters , from the video titled Enzymes (Updated) .
Amylase stands as a cornerstone enzyme in the realm of carbohydrate metabolism, orchestrating the breakdown of starch into simpler sugars. Its presence and activity are fundamental, not only to biological systems but also to a diverse array of industrial applications.
This section serves as an introduction to the multifaceted world of amylase, highlighting its indispensable role and setting the stage for a deeper exploration of its properties, functions, and significance.
Amylase: A Vital Enzyme for Carbohydrate Metabolism
Amylase’s primary function lies in catalyzing the hydrolysis of starch, a complex carbohydrate composed of glucose units linked together.
This enzymatic action breaks down starch into smaller, more readily usable sugars, such as maltose and glucose. These sugars then serve as crucial energy sources for various biological processes.
The efficiency of this process is critical for energy production and overall metabolic balance. Without amylase, our bodies would struggle to extract energy from starchy foods.
A Glimpse into History: The Discovery of Amylase
The discovery of amylase dates back to the early 19th century, marking a significant milestone in the field of enzyme research.
In 1833, French chemists Anselme Payen and Jean-François Persoz made a groundbreaking observation: a malt extract could facilitate the breakdown of starch.
This pivotal discovery led to the isolation of the first enzyme, which they termed "diastase," now recognized as amylase. Their work laid the foundation for our modern understanding of enzymatic activity.
Importance and Diverse Applications of Amylase
Amylase's importance transcends the boundaries of biological systems, extending its influence into a wide spectrum of industrial sectors.
In the food industry, amylase plays a crucial role in baking, brewing, and the production of sweeteners. It enhances the texture and flavor of baked goods.
In the brewing industry, it is essential for converting starch into fermentable sugars, the very foundation of alcoholic beverages.
Beyond the food industry, amylase finds applications in textile manufacturing, paper production, and even the development of biofuels. Its versatility underscores its significance in modern technology.
Amylase's catalytic prowess has made it an invaluable tool, driving efficiency and innovation across diverse industries. Its applications continue to expand as researchers explore its potential in new and emerging fields.
Starch Unveiled: Structure, Composition, and Gelatinization
Amylase stands as a cornerstone enzyme in the realm of carbohydrate metabolism, orchestrating the breakdown of starch into simpler sugars. Its presence and activity are fundamental, not only to biological systems but also to a diverse array of industrial applications. This understanding of amylase's interaction with starch necessitates a closer look into the complex world of starch itself, its intricate structure, and the transformative process of gelatinization.
The Dual Nature of Starch: Amylose and Amylopectin
Starch, the primary energy storage polysaccharide in plants, is not a singular entity. Instead, it is a sophisticated blend of two distinct glucose polymers: amylose and amylopectin.
Their relative proportions and structural nuances dictate the overall properties and behavior of starch in various applications.
Amylose, a linear polymer of glucose units linked by α-1,4-glycosidic bonds, forms helical structures in aqueous solutions.
This helical conformation is crucial for its interaction with iodine, leading to the characteristic deep blue color observed in the iodine-starch test.
Amylopectin, in contrast, is a highly branched polymer with α-1,6-glycosidic linkages occurring approximately every 20-25 glucose units along the α-1,4-linked backbone.
This branching architecture prevents the formation of ordered helical structures, resulting in a less intense reddish-brown color with iodine.
The ratio of amylose to amylopectin varies depending on the botanical source of the starch. For instance, common corn starch typically contains around 25% amylose and 75% amylopectin, while waxy corn starch is composed almost entirely of amylopectin. This variation significantly influences the texture, digestibility, and industrial applications of different starches.
The Physical Properties of Starch
Starch, in its native form, exists as discrete granules within plant cells. These granules are insoluble in cold water and exhibit a characteristic size and shape that is specific to the plant source.
The crystalline structure of starch granules, resulting from the ordered arrangement of amylose and amylopectin molecules, contributes to their insolubility and resistance to enzymatic degradation in the raw state.
Gelatinization: The Starch Transformation
Gelatinization is a critical phase transition that occurs when starch granules are heated in the presence of water. This process involves the disruption of the crystalline structure within the granules, leading to swelling and water absorption.
The Gelatinization Process Explained
As the temperature rises, the hydrogen bonds holding the starch molecules together weaken. Water molecules then penetrate the granule, causing it to swell dramatically.
This swelling results in an increase in viscosity and translucency of the starch suspension. The gelatinization temperature range is specific to the type of starch, typically falling between 60°C and 80°C.
Factors Influencing Gelatinization
Several factors influence the gelatinization process, including:
-
Starch concentration: Higher concentrations result in a more viscous paste.
-
Water availability: Adequate water is crucial for granule swelling.
-
Temperature: Gelatinization occurs within a specific temperature range, dependent on the starch source.
-
pH: Extreme pH levels can affect the rate and extent of gelatinization.
-
Presence of other ingredients: Sugars and fats can influence gelatinization by competing for water or interfering with granule swelling.
Gelatinization is a crucial step in many food processing applications, as it contributes to the texture, stability, and digestibility of starch-based products. Understanding this process is fundamental to controlling the functional properties of starch in food formulations.
Amylase Isoforms: Alpha, Beta, and Gamma – A Comparative Look
[Starch Unveiled: Structure, Composition, and Gelatinization Amylase stands as a cornerstone enzyme in the realm of carbohydrate metabolism, orchestrating the breakdown of starch into simpler sugars. Its presence and activity are fundamental, not only to biological systems but also to a diverse array of industrial applications. This understanding of...] the enzyme necessitates a closer examination of its various forms, each possessing unique characteristics and playing distinct roles in starch hydrolysis. Alpha, beta, and gamma amylases, while sharing a common function, exhibit significant differences in their mechanism of action, substrate specificity, and the products they yield. A comparative analysis of these isoforms is crucial for appreciating the versatility of amylase and its wide-ranging applications.
Unveiling the Amylase Trio: α-Amylase, β-Amylase, and γ-Amylase
The amylase family comprises three principal members: α-amylase (alpha-amylase), β-amylase (beta-amylase), and γ-amylase (gamma-amylase), also known as glucoamylase. Each isoform is characterized by its unique catalytic properties and mode of action on starch molecules.
α-Amylase, an endoamylase, catalyzes the random hydrolysis of α-1,4-glycosidic bonds within the starch molecule. This random cleavage results in a mixture of shorter oligosaccharides, including dextrins, as well as some glucose and maltose. α-Amylase is found in both plants and animals, most notably in saliva and pancreatic juice.
β-Amylase, an exoamylase, acts on the non-reducing ends of starch molecules, cleaving off two glucose units at a time to yield maltose. This enzyme is primarily found in plants, particularly in germinating seeds.
γ-Amylase, another exoamylase, hydrolyzes α-1,4-glycosidic bonds, as well as α-1,6-glycosidic bonds at branch points in amylopectin. It cleaves single glucose units from the non-reducing ends of the starch molecule. γ-Amylase, also known as glucoamylase, is commonly found in microorganisms, such as fungi and bacteria.
Deconstructing the Mechanisms: Substrate Specificity and Action
The specificity of each amylase isoform arises from differences in their active site structure and the way they interact with the starch substrate. α-Amylase's ability to cleave internal α-1,4-glycosidic bonds randomly contrasts with β-amylase's sequential removal of maltose units from the non-reducing ends. γ-Amylase's capacity to hydrolyze both α-1,4 and α-1,6-glycosidic bonds allows it to completely degrade starch into glucose.
The mechanism of action also differs in terms of stereochemistry. α-Amylase typically proceeds with retention of the anomeric configuration, while β-amylase operates with inversion. γ-Amylase, depending on its source, can exhibit either retention or inversion.
The Hydrolysis Products: Dextrins, Maltose, and Glucose
The end products of starch hydrolysis vary depending on the amylase isoform involved. α-Amylase produces a mixture of dextrins (oligosaccharides of varying chain length), as well as some maltose and glucose. β-Amylase primarily yields maltose, a disaccharide composed of two glucose units. γ-Amylase exclusively generates glucose, the simplest monosaccharide.
The presence of α-1,6-glycosidic bonds in amylopectin can hinder the complete degradation of starch by α- and β-amylases alone. Debranching enzymes, such as pullulanase, are often required to remove these branch points, allowing for more complete hydrolysis.
In summary, α-amylase, β-amylase, and γ-amylase represent a diverse group of enzymes with distinct specificities and catalytic mechanisms. The interplay of these isoforms ensures efficient starch digestion and provides a range of products tailored to specific biological and industrial needs.
Amylase in Human Digestion: From Saliva to the Small Intestine
Having explored the nuanced differences between amylase isoforms, it’s essential to trace the enzyme’s journey through the human digestive system. This section will focus on amylase's pivotal role, starting in the mouth and extending to the small intestine. This is where the bulk of starch digestion occurs.
Salivary Amylase: The Oral Phase of Starch Digestion
The digestive process begins in the mouth, where salivary amylase, also known as ptyalin, is secreted by the salivary glands. This enzyme initiates the breakdown of complex carbohydrates into simpler sugars.
Upon food ingestion, salivary amylase is released into the oral cavity. It immediately begins hydrolyzing α-1,4-glycosidic bonds in starch and glycogen. This initial enzymatic activity results in a mixture of smaller oligosaccharides.
However, the activity of salivary amylase is short-lived. Once the food bolus reaches the acidic environment of the stomach, the low pH denatures the enzyme, halting its digestive action. The churning action of the stomach further mixes the partially digested food with gastric juices. This sets the stage for the next phase of carbohydrate digestion.
Pancreatic Amylase: Continuing Digestion in the Small Intestine
The duodenum, the first section of the small intestine, marks a critical juncture. Here, pancreatic amylase takes over the digestive process. Pancreatic amylase, synthesized and secreted by the pancreas, is released into the small intestine. It continues the hydrolysis of starch that salivary amylase initiated.
The Pancreas: An Essential Digestive Organ
The pancreas is a vital organ that serves both endocrine and exocrine functions. Its exocrine function includes producing and secreting various digestive enzymes, including pancreatic amylase.
These enzymes are crucial for breaking down carbohydrates, proteins, and fats in the small intestine. The secretion of pancreatic amylase is carefully regulated. This ensures optimal digestion and nutrient absorption.
Mechanism of Action in the Small Intestine
Pancreatic amylase, like its salivary counterpart, targets the α-1,4-glycosidic bonds within starch molecules. This leads to the production of smaller oligosaccharides, including maltose, maltotriose, and α-dextrins.
The optimal pH for pancreatic amylase activity is slightly alkaline. The duodenal environment is effectively neutralized by bicarbonate ions secreted by the pancreas. This ensures that amylase can function efficiently.
Final Digestion and Absorption of Starch
While amylase plays a crucial role in breaking down starch, it cannot hydrolyze the α-1,6-glycosidic bonds present at the branch points of amylopectin. This is where other enzymes, such as α-dextrinase (also known as isomaltase), come into play.
α-Dextrinase, located on the brush border of the small intestine's epithelial cells, specifically cleaves these branch points. This results in the further breakdown of oligosaccharides into glucose. Glucose, a monosaccharide, can then be absorbed into the bloodstream.
Absorption of Glucose and Other Monosaccharides
The absorption of glucose and other monosaccharides occurs primarily in the small intestine. This is facilitated by specialized transport proteins on the surface of intestinal cells.
The sodium-glucose cotransporter 1 (SGLT1) is responsible for the active transport of glucose and galactose. Fructose, on the other hand, is transported via facilitated diffusion through the GLUT5 transporter.
Once inside the intestinal cells, monosaccharides are transported into the bloodstream via the GLUT2 transporter. From there, they are carried to various tissues throughout the body for energy production or storage.
Factors Influencing Amylase Activity: Optimizing Digestion and Industrial Processes
Having explored the nuanced differences between amylase isoforms, it’s essential to trace the enzyme’s journey through the human digestive system. This section will focus on amylase's pivotal role, starting in the mouth and extending to the small intestine. This is where the bulk of starch digestion happens, but numerous factors can either enhance or impede amylase's effectiveness.
Understanding these factors is critical for optimizing both natural digestive processes and industrial applications where amylase is employed. We must consider how enzyme kinetics, the fundamental mechanism of hydrolysis, and environmental conditions like pH and temperature contribute to amylase's overall performance.
Enzyme Kinetics and Starch Breakdown
Enzyme kinetics describes the rate at which enzymes catalyze reactions. For amylase, this translates to how quickly it breaks down starch into simpler sugars.
The Michaelis-Menten model provides a foundational understanding. This model describes the relationship between substrate concentration (starch) and reaction velocity.
At low starch concentrations, the reaction rate increases almost linearly with increasing starch. However, as starch concentration increases, the reaction rate plateaus, approaching a maximum velocity (Vmax).
This is because the enzyme's active sites become saturated with substrate.
The Michaelis constant (Km) reflects the substrate concentration at which the reaction rate is half of Vmax. Km is a measure of the enzyme's affinity for its substrate; a lower Km indicates a higher affinity. Understanding these kinetic parameters allows for fine-tuning conditions for optimal starch breakdown.
The Hydrolysis Reaction Mechanism
Amylase catalyzes the hydrolysis of α-1,4-glycosidic bonds in starch. This is achieved through a nucleophilic attack.
The oxygen atom in a water molecule acts as a nucleophile, attacking the carbon atom in the glycosidic bond. This breaks the bond, adding a hydroxyl group (-OH) to one glucose residue and a hydrogen atom (-H) to the other.
This process requires the precise positioning of the starch molecule within the enzyme's active site, which facilitates the reaction. The specific amino acid residues within the active site play a crucial role in stabilizing the transition state and lowering the activation energy of the reaction.
Environmental Factors Affecting Amylase Activity
Several environmental factors significantly impact amylase's efficiency:
-
pH Levels: Amylase exhibits optimal activity within a specific pH range. Salivary amylase functions best at a slightly acidic to neutral pH (around 6.7-7.0). Pancreatic amylase operates effectively in a slightly alkaline environment (around pH 7.0-7.5). Deviations from these optimal ranges can alter the enzyme's structure and reduce its catalytic activity. Extreme pH levels can lead to denaturation, rendering the enzyme completely inactive.
-
Temperature: Temperature also plays a critical role in amylase activity. The enzyme's activity typically increases with temperature, up to an optimum point. Beyond this point, the enzyme begins to denature. The optimal temperature for many amylases is between 30°C and 40°C.
-
Inhibitors: Certain molecules can inhibit amylase activity. Some inhibitors bind to the active site, preventing substrate binding (competitive inhibition). Others bind to a different site on the enzyme, altering its shape and reducing its activity (non-competitive inhibition). For instance, certain metal ions or specific chemicals can act as amylase inhibitors.
Optimizing these factors is crucial for maximizing amylase activity. In industrial settings, careful control of pH, temperature, and the absence of inhibitors are essential for efficient starch processing. In the context of human digestion, maintaining a healthy gut environment supports optimal amylase function and efficient carbohydrate metabolism.
Amylase Beyond Humans: Plants and Microorganisms as Amylase Sources
Having explored the nuanced differences between amylase isoforms, it’s essential to trace the enzyme’s journey through the human digestive system. This section will focus on amylase's pivotal role, starting in the mouth and extending to the small intestine. This is...
...not quite accurate. Instead, let's shift our focus outwards, beyond the human body. While we often consider amylase within the context of human digestion and industrial processes, this starch-degrading enzyme plays critical roles in a vast array of organisms, notably in the plant kingdom and among microorganisms. Investigating these diverse sources not only enriches our understanding of amylase's biological significance but also unveils novel avenues for its application.
Amylase in the Plant Kingdom: A Seed's First Nourishment
Amylase is far from solely an animal enzyme. Plants rely on it heavily, especially during seed germination.
Stored within the seed is a wealth of starch, a critical energy reserve for the nascent plant. Amylase is the key that unlocks this reserve, breaking down the complex starch molecules into simpler sugars like glucose and maltose.
These sugars provide the essential fuel for the seedling to sprout, develop roots, and begin photosynthesis.
The production of amylase is tightly regulated during germination, triggered by hormonal signals and environmental cues. This ensures that the seedling has access to the energy it needs at precisely the right time.
Furthermore, different plant species exhibit variations in the types and amounts of amylase produced, reflecting their specific germination strategies and energy requirements. Understanding these variations could provide insights into optimizing crop yields and improving seed quality.
Microbial Amylases: A Treasure Trove of Industrial Potential
Microorganisms, including bacteria and fungi, represent a particularly rich source of amylase. Unlike animals that primarily use amylase for digestion, many microbes secrete amylase into their environment to break down starch for their own nutritional needs.
This extracellular secretion makes microbial amylases readily accessible for industrial applications.
The advantages of using microbial amylases are numerous: they can be produced in large quantities through fermentation, are often more stable and active under a wider range of conditions than plant or animal amylases, and can be genetically modified to enhance their properties.
Bacterial Amylases: Workhorses of the Starch Industry
Bacteria such as Bacillus subtilis and Bacillus amyloliquefaciens are widely used for industrial production of α-amylase.
These bacterial amylases are particularly valued for their thermostability, allowing them to function at high temperatures, crucial in many industrial processes like starch liquefaction. They are also easily cultivated at an industrial scale.
Fungal Amylases: Tailored for Specific Applications
Fungi, notably Aspergillus niger and Rhizopus oryzae, are another important source of amylase. Fungal amylases often exhibit unique specificities and are particularly well-suited for applications such as baking, where they contribute to bread texture and flavor.
Moreover, fungal amylases are often used in the production of various syrups and sweeteners.
Genetic Engineering: Optimizing Microbial Amylases for Enhanced Performance
The power of genetic engineering allows scientists to further enhance the properties of microbial amylases. By modifying the genes encoding amylase, researchers can improve their thermostability, pH tolerance, substrate specificity, and overall activity.
These engineered amylases are increasingly used in a variety of industrial processes, contributing to greater efficiency and sustainability. The ability to tailor these enzymes to specific applications makes them invaluable tools in modern biotechnology.
Industrial Applications of Amylase: From Food to Fuel
Following our exploration of amylase production beyond the human body, it becomes evident that this enzyme is not just a digestive aid; it’s a linchpin in a multitude of industrial processes. The versatility of amylase stems from its ability to efficiently break down starch into simpler sugars, a transformation that underpins numerous applications spanning the food, beverage, and biofuel industries. This section will explore these diverse applications, underscoring the enzyme's pivotal role in modern industrial practices.
Amylase in the Food Industry: A Versatile Catalyst
Amylase's influence within the food industry is pervasive, enhancing various processes from baking to sweetener production. Its ability to degrade starch into smaller, more manageable sugar molecules unlocks desirable qualities in final products.
Baking: Enhancing Texture and Shelf Life
In baking, amylases are added to dough to break down starch into sugars. This provides a readily available food source for yeast, accelerating fermentation and increasing the volume and texture of baked goods. The resulting sugars also contribute to the Maillard reaction, enhancing crust color and flavor. Furthermore, amylase can improve the shelf life of bread by reducing staling, keeping it softer for longer.
Syrup Production: Creating Sweeteners from Starch
Amylases play a crucial role in the production of corn syrup and other sweeteners. Starch from corn or other sources is hydrolyzed using amylase to produce a mixture of glucose, maltose, and other sugars.
This process allows for the creation of high-fructose corn syrup (HFCS), a widely used sweetener in the food and beverage industry. The precise control offered by enzymatic hydrolysis allows manufacturers to tailor the sweetness profile of the syrup to specific applications.
Brewing Industry: The Alchemist of Fermentation
The brewing industry relies heavily on amylases to convert starch into fermentable sugars, which are then consumed by yeast to produce alcohol.
The Mashing Process: Releasing Sugars for Fermentation
During the mashing process, malted barley (or other grains) is mixed with hot water to activate naturally occurring amylases. These enzymes break down the starches in the grain into maltose and other simple sugars.
This process is crucial for creating a "wort" rich in fermentable sugars, the foundation for alcoholic beverages. The precise temperature and duration of mashing are carefully controlled to optimize enzyme activity and ensure a desirable sugar profile for fermentation.
Adjunct Grains and Supplemental Enzymes
Many breweries utilize adjunct grains, such as corn or rice, to supplement the starch content of their mash. These grains often require the addition of exogenous amylases to ensure complete starch conversion, particularly in the production of light-bodied beers. Supplemental amylases can also be used to further break down complex carbohydrates, creating drier, more attenuated beers with lower residual sweetness.
Clinical Significance of Amylase: Diagnosing Pancreatic Disorders
Following our exploration of amylase production beyond the human body, it becomes evident that this enzyme is not just a digestive aid; it’s a linchpin in a multitude of industrial processes. The versatility of amylase stems from its ability to efficiently break down starch into simpler sugars, a capability that also makes it a crucial diagnostic marker in clinical settings, particularly for pancreatic disorders. Analyzing amylase levels in bodily fluids provides invaluable insights into the health and functionality of the pancreas, an organ vital for both digestion and endocrine regulation.
Amylase as a Diagnostic Marker
Serum amylase levels are routinely measured to assess pancreatic function and to diagnose conditions such as pancreatitis. Elevated amylase levels often indicate pancreatic inflammation or injury, while abnormally low levels can suggest chronic pancreatic damage or, in rare cases, genetic mutations affecting amylase production. The interpretation of these levels, however, requires careful consideration of the patient's clinical history and other diagnostic findings.
Acute Pancreatitis: A Rapid Rise in Amylase
One of the most common clinical applications of amylase testing is in the diagnosis of acute pancreatitis.
In this condition, the pancreas becomes acutely inflamed, leading to the release of large amounts of amylase into the bloodstream. Serum amylase levels typically rise rapidly within a few hours of the onset of symptoms, often reaching several times the upper limit of the normal range.
It is important to note, however, that amylase levels do not always correlate directly with the severity of pancreatitis. Some patients with severe pancreatitis may have only moderately elevated amylase levels, while others with milder forms of the disease may exhibit very high levels.
Other factors, like kidney function, can influence serum amylase concentrations, warranting comprehensive clinical evaluation.
The Role of Lipase
While amylase is a useful marker for acute pancreatitis, it is not always the most reliable. Lipase, another pancreatic enzyme, is often considered a more specific and sensitive marker for this condition.
Lipase levels tend to remain elevated for a longer period than amylase levels, making it a more useful diagnostic tool, especially if there is a delay between the onset of symptoms and the time of testing. In clinical practice, both amylase and lipase levels are often measured together to improve the accuracy of diagnosis.
Chronic Pancreatitis: A More Complex Picture
In contrast to acute pancreatitis, chronic pancreatitis presents a more complex diagnostic challenge with regards to amylase levels. In chronic pancreatitis, the pancreas undergoes gradual and irreversible damage, leading to a progressive loss of function.
In the early stages of the disease, amylase levels may be normal or only mildly elevated. However, as the disease progresses and more pancreatic tissue is destroyed, the pancreas may lose its ability to produce and secrete amylase. In these later stages, amylase levels may actually be low or even undetectable.
Therefore, normal or low amylase levels do not necessarily rule out chronic pancreatitis. Imaging studies, such as CT scans or MRI, and other pancreatic function tests are often needed to confirm the diagnosis.
Other Conditions Affecting Amylase Levels
It's important to recognize that elevated amylase levels are not always indicative of pancreatic disease. Other conditions, such as:
- Salivary gland disorders (e.g., mumps)
- Bowel obstruction
- Kidney failure
- Certain medications
Can also cause elevated serum amylase.
Furthermore, macroamylasemia, a benign condition in which amylase forms complexes with immunoglobulins, can result in persistently elevated amylase levels without any underlying pathology. A thorough clinical evaluation is essential to differentiate these conditions from pancreatic disorders.
Isoamylase Testing: Refining the Diagnosis
To improve the specificity of amylase testing, isoamylase assays can be used to differentiate between pancreatic amylase (P-amylase) and salivary amylase (S-amylase).
This can be particularly helpful in cases where the source of elevated amylase is unclear. For example, in patients with suspected pancreatitis who also have salivary gland disease, isoamylase testing can help determine whether the elevated amylase is coming from the pancreas or the salivary glands.
This level of specificity offers clinicians a refined diagnostic capability, allowing for better targeted treatment strategies.
In conclusion, amylase remains a valuable tool in the diagnosis of pancreatic disorders, particularly acute pancreatitis. However, it is essential to interpret amylase levels in the context of the patient's overall clinical presentation and to consider other diagnostic modalities, such as lipase testing and imaging studies. A comprehensive and thoughtful approach is crucial for accurate diagnosis and optimal patient care.
Measuring Amylase Activity: Enzyme Assays Explained
Following our exploration of amylase production beyond the human body, it becomes evident that this enzyme is not just a digestive aid; it’s a linchpin in a multitude of industrial processes. The versatility of amylase stems from its ability to efficiently break down starch into simpler sugars. Quantifying precisely how much amylase is present and how actively it's functioning is crucial for a wide array of applications, from diagnosing medical conditions to optimizing industrial bioprocesses. This section delves into the methodologies employed to measure amylase activity, shedding light on the principles behind these essential enzyme assays.
Understanding Enzyme Assays
Enzyme assays are the cornerstone of biochemical research and clinical diagnostics. They provide a quantitative measure of enzyme activity, reflecting the rate at which an enzyme catalyzes a specific reaction. For amylase, this means measuring the rate at which it breaks down starch into smaller sugar molecules.
The key principle behind these assays is to monitor the change in substrate (starch) or the appearance of product (sugars) over time. The rate of this change is directly proportional to the amount of active amylase present in the sample.
Types of Amylase Assays
Several methods are available for measuring amylase activity, each with its own advantages and limitations. These methods can be broadly categorized into amyloclastic and saccharogenic assays, along with more modern, chromogenic, and enzymatic approaches.
Amyloclastic Assays
Amyloclastic assays are based on measuring the decrease in starch concentration as it is hydrolyzed by amylase. The most common of these is the iodine-starch method. Starch forms a complex with iodine, resulting in a characteristic blue color. As amylase breaks down the starch, the intensity of the blue color diminishes. The rate of color decrease is then correlated with amylase activity.
This method is relatively simple and inexpensive, making it suitable for high-throughput screening. However, it is less precise compared to other methods and can be affected by the presence of interfering substances.
Saccharogenic Assays
Saccharogenic assays, conversely, focus on measuring the increase in reducing sugars produced as a result of starch hydrolysis. This is often accomplished using the Somogyi-Nelson method, which relies on the reduction of copper ions by reducing sugars. The reduced copper then reacts with a reagent to form a colored product that can be measured spectrophotometrically.
While saccharogenic assays are generally more sensitive than amyloclastic assays, they can be more complex and time-consuming. Furthermore, the presence of other reducing substances in the sample can lead to inaccurate results.
Chromogenic Assays
Chromogenic assays employ substrates that release a colored product upon hydrolysis by amylase. A common example involves the use of p-nitrophenyl-α-D-maltoside (PNPG2) as a substrate. Amylase cleaves PNPG2 to release p-nitrophenol, a yellow-colored compound that can be measured spectrophotometrically.
These assays are highly sensitive and specific, making them ideal for measuring low levels of amylase activity. They are also relatively simple to perform and can be easily automated.
Enzymatic Assays
Enzymatic assays involve coupling the amylase reaction to a series of enzymatic reactions that ultimately produce a measurable product. For instance, the glucose produced by amylase can be quantified using glucose oxidase and peroxidase, resulting in a color change that is proportional to amylase activity.
These assays offer excellent specificity and sensitivity, but they can be more expensive due to the need for additional enzymes and reagents.
Factors Affecting Amylase Assay Accuracy
Regardless of the method used, several factors can influence the accuracy of amylase assays:
-
Temperature: Amylase activity is highly temperature-dependent. Assays should be performed at a controlled temperature, typically 37°C for clinical samples, to ensure accurate results.
-
pH: Amylase activity is also pH-dependent, with optimal activity occurring within a specific pH range. The pH of the assay buffer should be carefully controlled to maintain optimal enzyme activity.
-
Substrate Concentration: The concentration of starch in the assay mixture can affect the measured amylase activity. It is important to use a substrate concentration that is saturating for the enzyme.
-
Inhibitors: The presence of inhibitors in the sample can reduce amylase activity and lead to falsely low results.
Applications of Amylase Activity Measurement
Measuring amylase activity has wide-ranging applications across various fields:
-
Clinical Diagnostics: As mentioned earlier, amylase activity is routinely measured in serum and urine to diagnose pancreatic disorders such as pancreatitis and pancreatic cancer.
-
Food Industry: Amylase assays are used to monitor the activity of amylases in food processing, such as baking and brewing.
-
Biotechnology: Amylase activity is measured to optimize enzyme production and purification processes.
-
Research: Amylase assays are used to study the properties of amylases and to investigate their role in various biological processes.
In conclusion, accurately measuring amylase activity is critical for a diverse array of applications. From diagnosing diseases to optimizing industrial processes, a thorough understanding of enzyme assays and their limitations is essential for obtaining reliable and meaningful results.
Video: What Enzyme Breaks Down Starch? Amylase Guide
Frequently Asked Questions About Amylase and Starch
Where is amylase produced in the body?
Amylase, the enzyme that breaks down starch, is primarily produced in two places: the salivary glands in your mouth and the pancreas. The amylase produced in the salivary glands begins the digestive process in the mouth, while pancreatic amylase continues it in the small intestine.
What types of starches can amylase digest?
Amylase is effective at breaking down various types of starches, including amylose and amylopectin. These are the two main polysaccharides found in plant-based foods. Essentially, what enzyme breaks down starch does so regardless of its form, converting it into simpler sugars.
What happens if I don't have enough amylase?
If your body doesn't produce enough amylase, you may experience difficulty digesting starches. This can lead to symptoms such as bloating, diarrhea, and abdominal discomfort after eating carbohydrate-rich meals. Properly functioning amylase is crucial for breaking down starch into usable energy.
Is there a medical test to check amylase levels?
Yes, a blood test can measure your amylase levels. This test is often used to help diagnose conditions affecting the pancreas, such as pancreatitis. Elevated or decreased amylase levels can indicate problems with your pancreatic function and its ability to produce what enzyme breaks down starch.
So, there you have it! Hopefully, you now have a better understanding of amylase, the amazing enzyme that breaks down starch. Next time you're enjoying a starchy snack, remember the microscopic workhorses in your body that are making it all possible!