Fusion Chemistry: The Ultimate Guide You Need to Read
The transformative potential of fusion chemisty is revolutionizing numerous fields, and this guide is your comprehensive resource. The Lawrence Livermore National Laboratory plays a pivotal role in advancing fusion chemisty research. Understanding the principles of nuclear fusion is foundational to grasping the intricacies of this discipline. Sophisticated spectroscopy techniques are instrumental for analyzing the products of fusion chemisty. This article provides an in-depth exploration of fusion chemisty's core concepts and applications, making it the ultimate guide you need to read.
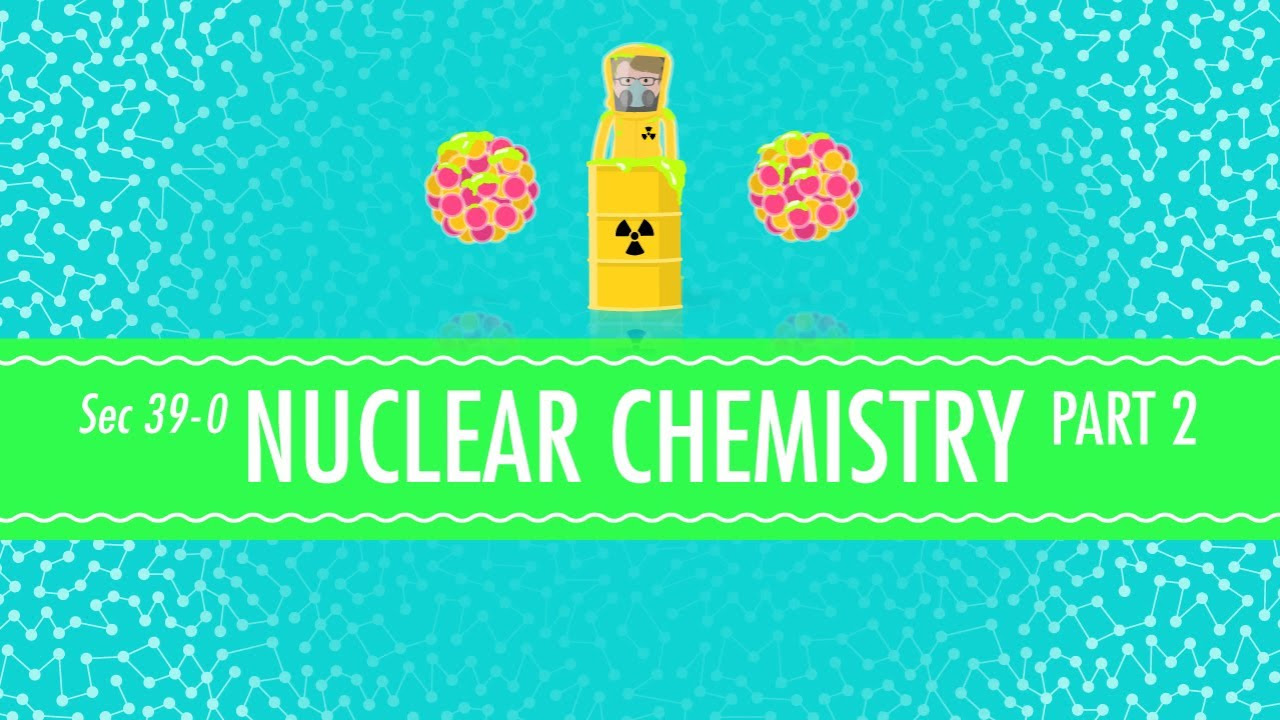
Image taken from the YouTube channel CrashCourse , from the video titled Nuclear Chemistry Part 2 - Fusion and Fission: Crash Course Chemistry #39 .
The relentless quest for a clean, sustainable, and virtually inexhaustible energy source has led scientists and engineers to explore the fascinating realm of fusion chemistry. This field, at the intersection of nuclear physics and chemical engineering, holds the tantalizing promise of replicating the power of the stars here on Earth.
Defining Nuclear Fusion in Chemical Terms
At its core, nuclear fusion is a nuclear reaction where two or more atomic nuclei combine to form a single, heavier nucleus. This process is accompanied by the release of a tremendous amount of energy, governed by Einstein's famous equation, E=mc².
From a chemical perspective, fusion involves the manipulation of isotopes of light elements, primarily hydrogen, to overcome their natural electrostatic repulsion. This requires extreme conditions of temperature and pressure, mimicking those found in the sun's core. The resulting energy release is far greater than that of any chemical reaction, making it an exceptionally potent energy source. Unlike nuclear fission, fusion does not produce long-lived radioactive waste products, further enhancing its appeal.
The Global Energy Crisis: A Call for Fusion
The world is grappling with an unprecedented energy crisis, driven by escalating demand, dwindling fossil fuel reserves, and the urgent need to mitigate climate change. Our reliance on fossil fuels has led to significant environmental consequences, including greenhouse gas emissions, air and water pollution, and ecological damage.
Renewable energy sources like solar and wind are playing an increasingly important role, but their intermittent nature and land-use requirements pose challenges to widespread adoption. Nuclear fission, while providing a low-carbon energy source, faces concerns regarding reactor safety and nuclear waste disposal.
Fusion energy offers a compelling alternative, promising a secure, sustainable, and environmentally responsible energy supply. The fuel for fusion, primarily deuterium, is abundant in seawater, ensuring a virtually unlimited resource. By harnessing the power of fusion, we can drastically reduce our dependence on fossil fuels and mitigate the worst effects of climate change.
Fusion Chemistry: A Sustainable Future
The potential benefits of fusion chemistry extend far beyond addressing the energy crisis. A successful fusion energy program would revolutionize various sectors, including transportation, manufacturing, and medicine.
Clean and abundant energy would enable the production of synthetic fuels, powering vehicles and aircraft without contributing to air pollution. Advanced materials could be developed, leading to more efficient and sustainable manufacturing processes. Medical isotopes, essential for diagnosis and treatment, could be produced on a large scale, improving healthcare outcomes.
Fusion chemistry represents a paradigm shift in energy production, offering a path towards a truly sustainable and prosperous future for all. While significant technical challenges remain, the potential rewards are too great to ignore. Continued investment in fusion research and development is crucial to unlocking its full potential and realizing the promise of a clean, abundant, and sustainable energy future.
The global energy crisis necessitates exploring revolutionary solutions, and nuclear fusion stands out as a beacon of hope. But before we can unlock the stars' potential, it's crucial to understand the chemical underpinnings of this powerful reaction.
The Chemistry of Fusion: Core Concepts Explained
The allure of fusion lies in its promise of abundant, clean energy. To grasp this promise, we must delve into the fundamental chemical principles that govern fusion reactions. This involves understanding the unique properties of fusion fuels, the nature of plasma, and the conditions necessary for sustained energy production.
Deuterium and Tritium: The Ideal Fusion Fuels
At the heart of most fusion research lies the reaction between two isotopes of hydrogen: deuterium (D) and tritium (T). Deuterium, with one proton and one neutron, is readily available in seawater. Tritium, with one proton and two neutrons, is rarer but can be produced from lithium.
Why these two isotopes?
Their nuclear structure makes the D-T reaction the most accessible pathway to fusion, requiring relatively lower temperatures and yielding a significant energy output.
The reaction produces helium and a neutron, along with a massive release of energy.
Isotopic Properties and Fusion Reactivity
The key to deuterium and tritium's suitability lies in their isotopic properties. The addition of neutrons to the hydrogen nucleus alters the strong nuclear force, influencing the energy landscape of the fusion reaction.
This results in a lower energy barrier for fusion compared to other elements.
This lower barrier translates to a more achievable reaction rate at attainable temperatures. Furthermore, the products of the D-T reaction are relatively benign: helium, an inert gas, and a neutron that can be used to breed more tritium.
Sourcing, Handling, and Storage
Deuterium, found naturally in seawater at a concentration of about 0.015%, can be extracted through electrolysis or chemical exchange processes.
Tritium, being radioactive with a half-life of about 12.3 years, is not naturally abundant. It's primarily produced through neutron bombardment of lithium in a nuclear reactor.
The handling and storage of tritium present unique chemical challenges. Due to its radioactivity and ability to permeate materials, tritium must be contained within specialized, leak-tight systems.
Chemical forms of tritium, such as tritiated water (HTO), require careful management to prevent environmental release.
Harnessing the Power of Plasma: The Fourth State of Matter
Fusion reactions don't occur under ordinary conditions. The positively charged nuclei of deuterium and tritium repel each other electrostatically. To overcome this repulsion, the fuel must be heated to extreme temperatures, on the order of millions of degrees Celsius, transforming it into plasma.
Plasma, often called the fourth state of matter, is an ionized gas where electrons are stripped from atoms, creating a mixture of ions and free electrons.
In this state, the nuclei have enough kinetic energy to overcome their electrostatic repulsion and fuse.
Creating and Maintaining Plasma
Creating and maintaining plasma requires a constant input of energy. This can be achieved through various methods, including radio frequency heating, microwave heating, and neutral beam injection.
The challenge lies in sustaining the plasma at the required temperature and density for a sufficient duration to achieve net energy gain.
Plasma Confinement Techniques
Containing plasma at these extreme temperatures is a formidable challenge. Any contact with the reactor walls would instantly cool the plasma, halting the fusion reaction. Therefore, sophisticated confinement techniques are essential.
Two primary approaches are being explored: magnetic confinement and inertial confinement.
Magnetic confinement uses strong magnetic fields to trap the charged particles of the plasma, preventing them from colliding with the reactor walls.
Inertial confinement involves compressing and heating a small fuel pellet using lasers or particle beams, creating a brief but intense burst of fusion reactions.
From a chemical perspective, understanding the behavior of plasma requires considering its unique properties: high electrical conductivity, sensitivity to magnetic fields, and the complex interplay of ionization, recombination, and radiation processes.
Achieving Controlled Nuclear Fusion: A Delicate Balance
Achieving controlled nuclear fusion is not just about reaching high temperatures and densities; it's about maintaining those conditions long enough for the fusion reactions to produce more energy than is required to sustain the plasma.
Demystifying the Lawson Criterion
The Lawson criterion is a key metric that defines the conditions necessary for achieving sustained fusion. It states that the product of the plasma density (n), the confinement time (τ), and the ion temperature (T) must exceed a certain value for net energy gain.
This criterion highlights the delicate balance required for successful fusion. Increasing the density, confinement time, or temperature can all contribute to achieving the Lawson criterion.
Optimizing Reaction Rates
Optimizing reaction rates involves carefully controlling the temperature, pressure, and density of the plasma. Higher temperatures increase the kinetic energy of the ions, leading to more frequent and energetic collisions.
Higher densities increase the probability of collisions. However, exceeding certain limits can lead to instability and plasma disruptions.
Maintaining the optimal balance requires sophisticated control systems and a deep understanding of plasma physics and chemistry.
The lower barrier translates to a more achievable reaction rate at the extreme temperatures required for fusion. The physics are compelling, but the engineering hurdles remain significant. That's why scientists are exploring diverse methods to bring fusion energy to fruition.
Major Approaches to Fusion Chemistry: Magnetic vs. Inertial Confinement
The quest for controlled nuclear fusion has led to the development of two major approaches: magnetic confinement and inertial confinement.
Each method tackles the challenge of creating and sustaining the extreme conditions necessary for fusion in fundamentally different ways. Understanding these approaches, their scientific underpinnings, and the engineering challenges they present is crucial for evaluating the progress and potential of fusion energy.
Magnetic Confinement: Taming Fusion with Magnetic Fields
Magnetic confinement fusion (MCF) relies on the principle of using powerful magnetic fields to contain and control the superheated plasma in which fusion reactions occur. Since the electrically charged plasma particles are forced to follow the magnetic field lines, this prevents the plasma from contacting and damaging the reactor walls.
The Tokamak Design and its Chemical Implications
The Tokamak is the most widely researched MCF device. Its donut shape (torus) creates a helical magnetic field that confines the plasma.
Powerful magnets, arranged around the torus, generate this field.
From a chemical perspective, the Tokamak presents several unique challenges. The plasma, composed of ionized deuterium and tritium, interacts intensely with the materials of the reactor walls, even with the magnetic confinement.
Controlling these interactions is crucial to prevent contamination of the plasma and damage to the reactor.
The choice of materials for the reactor walls is therefore a key chemical consideration, with research focusing on materials like beryllium, tungsten, and advanced steels that can withstand the extreme temperatures and radiation.
Furthermore, the process of fueling the plasma with deuterium and tritium and extracting the helium "ash" produced by the fusion reaction requires careful chemical control and management.
ITER: A Global Collaboration
ITER (International Thermonuclear Experimental Reactor) represents the most ambitious MCF project to date. This international collaboration brings together scientists and engineers from around the world to build a Tokamak capable of producing a self-sustaining fusion reaction – often referred to as a "burning plasma."
The chemical implications of ITER are immense.
ITER requires advanced materials science and engineering to build a reactor capable of withstanding the harsh conditions of a burning plasma.
It also necessitates the development of sophisticated fuel-cycle technologies to handle the large quantities of deuterium and tritium needed for sustained fusion.
ITER will serve as a crucial testbed for validating the scientific and technological feasibility of MCF and paving the way for future commercial fusion power plants.
Inertial Confinement: Compressing Fuel with Laser Power
Inertial confinement fusion (ICF) takes a different approach. Instead of using magnetic fields, ICF relies on the rapid compression of a small fuel pellet to achieve the conditions necessary for fusion.
This compression is typically achieved using powerful lasers or particle beams that rapidly heat the surface of the pellet, causing it to implode.
The National Ignition Facility (NIF)
The National Ignition Facility (NIF) at Lawrence Livermore National Laboratory is the leading ICF research facility in the United States.
NIF uses 192 high-energy lasers to compress a tiny capsule containing deuterium and tritium.
The goal is to achieve "ignition," a state where the energy released by the fusion reactions is greater than the energy used to initiate the compression, resulting in a self-sustaining fusion burn.
The Chemistry of Inertial Confinement Fusion
The chemistry of ICF is centered on the precise control of laser-matter interactions and fuel compression. The fuel pellet itself is a carefully engineered sphere of deuterium and tritium, often frozen to increase its density.
The composition and structure of the pellet are crucial to achieving uniform compression and avoiding instabilities that can disrupt the fusion reaction.
The lasers must be precisely tuned and focused to deliver energy efficiently to the pellet surface, creating a uniform implosion. The ablation of the outer layers of the pellet creates a rocket-like effect, driving the inner layers inward and compressing the fuel to extremely high densities and temperatures.
Understanding the complex plasma physics and hydrodynamics of this process requires sophisticated computer simulations and experimental diagnostics.
The development of advanced target materials and laser technologies is critical to improving the efficiency and stability of ICF and ultimately achieving ignition.
Chemical Challenges in Fusion Reactors: Materials and Fuel Cycle
While the promise of limitless, clean energy from fusion is tantalizing, the path to realizing this vision is paved with formidable chemical engineering challenges. Building and operating practical fusion reactors demands innovative solutions in materials science and the management of the fuel cycle. These are not mere engineering details; they are fundamental chemical hurdles that must be overcome to achieve sustainable fusion power.
Materials Science and Engineering: Building Robust Fusion Reactors
The interior of a fusion reactor is an extraordinarily hostile environment. Materials must withstand temperatures hotter than the sun, intense neutron radiation, and extreme pressures. These conditions can degrade and weaken structural components, leading to failures and shutdowns.
Extreme Temperatures and Pressures
Imagine a vessel where the temperature soars to hundreds of millions of degrees Celsius. The extreme heat, coupled with the pressure exerted by the plasma, places immense stress on the reactor's walls. Conventional materials simply cannot withstand this onslaught without melting, warping, or failing catastrophically.
Radiation Resistance: A Critical Factor
The high-energy neutrons produced during fusion reactions bombard the reactor materials, causing atomic displacements and the formation of defects in the crystal structure. Over time, this radiation damage leads to embrittlement, swelling, and reduced thermal conductivity, ultimately compromising the reactor's structural integrity.
Developing radiation-resistant materials is therefore paramount. Researchers are exploring advanced alloys, ceramics, and composites designed to minimize radiation damage and maintain their mechanical properties over extended periods. This requires a deep understanding of the interaction between radiation and matter at the atomic level.
Current research is focused on:
- Reduced-activation ferritic/martensitic steels, which exhibit good resistance to swelling and embrittlement.
- Tungsten alloys, prized for their high melting point and ability to withstand intense heat fluxes.
- Silicon carbide composites, offering excellent high-temperature strength and radiation resistance.
Fuel Cycle Management: Handling and Recycling Fusion Fuels
A sustainable fusion power plant requires a closed fuel cycle, where the fuel is efficiently recycled and any radioactive waste is managed responsibly. This involves several key processes.
Separating and Purifying Fusion Fuels
The exhaust gas from a fusion reactor contains a mixture of deuterium, tritium, helium (the fusion product), and other impurities. Separating and purifying the deuterium and tritium for reuse is essential to minimize fuel consumption and reduce the risk of radioactive releases.
Cryogenic distillation is a commonly used technique for separating these isotopes based on their slightly different boiling points. Advanced membrane technologies are also being developed to improve the efficiency and reduce the cost of this process.
Lithium Blankets: Breeding Tritium and Managing Waste
Tritium is a radioactive isotope of hydrogen that is relatively scarce in nature. To ensure a sustainable fuel supply, fusion reactors are designed to breed tritium from lithium.
A lithium blanket, surrounding the reactor core, absorbs neutrons produced during fusion reactions. These neutrons react with lithium to produce tritium and helium. The tritium is then extracted from the blanket and recycled back into the reactor.
The lithium blanket also plays a crucial role in managing radioactive waste. By absorbing neutrons, it can transmute long-lived radioactive isotopes into shorter-lived or stable isotopes, reducing the overall burden of nuclear waste disposal.
Fusion Power Plant : A Comprehensive Overview
A fusion power plant integrates the complex chemical processes of plasma confinement, heat extraction, and fuel cycle management into a functional energy-generating system. Understanding how these elements work together is crucial for appreciating the potential and the challenges of fusion energy.
How a Fusion Power Plant Works
The basic principle involves using the heat generated by fusion reactions to produce steam, which drives turbines and generates electricity, similar to conventional power plants. However, the unique aspects of fusion introduce several key differences:
- Plasma Confinement: Magnetic or inertial confinement systems create the conditions for fusion.
- Heat Extraction: A coolant, typically water or helium, circulates through the reactor to absorb the intense heat generated by the plasma.
- Tritium Breeding: Lithium blankets produce tritium fuel from neutron interactions.
- Power Conversion: The heat is used to generate steam, which drives turbines and generates electricity.
The Impact of Fusion Reactors
If successfully developed, fusion reactors have the potential to dramatically alter the energy landscape. Fusion offers a clean, abundant, and sustainable energy source that could mitigate climate change and ensure energy security for future generations.
- Reduced greenhouse gas emissions
- Elimination of long-lived nuclear waste
- Abundant fuel resources
- Enhanced energy security
Despite these tremendous potential, significant challenges remain in materials science, fuel cycle management, and reactor design. Overcoming these chemical hurdles is critical for transforming fusion from a scientific dream into a practical reality.
Potential of Helium-3 in Fusion Chemistry: A Futuristic Fuel
While deuterium and tritium currently headline fusion fuel discussions, the allure of Helium-3 (He-3) simmers beneath the surface, promising a potentially cleaner and more efficient fusion pathway. This section explores the theoretical advantages of He-3 fusion while acknowledging the significant, and perhaps insurmountable, obstacles that hinder its widespread adoption.
The Allure of Aneutronic Fusion
The primary advantage of Helium-3 lies in its potential for aneutronic fusion. When He-3 fuses with deuterium (D), the primary reaction produces Helium-4 (He-4, a stable isotope) and a proton (p+):
He-3 + D → He-4 + p+
Unlike deuterium-tritium (D-T) fusion, which releases a substantial portion of its energy as high-energy neutrons, the D-He-3 reaction primarily produces charged particles.
These charged particles offer several key benefits:
-
Reduced Radioactivity: The absence of high-energy neutrons significantly reduces neutron activation of reactor materials, leading to lower levels of radioactive waste and easier reactor maintenance.
-
Direct Energy Conversion: Charged particles can be directly converted into electricity using advanced energy conversion technologies, potentially increasing overall efficiency compared to traditional thermal energy conversion methods.
-
Smaller Reactor Size: Reduced neutron flux simplifies reactor shielding requirements, potentially enabling the construction of smaller and more compact fusion reactors.
Helium-3: A Cleaner, More Efficient Future?
These advantages paint a compelling picture of a future powered by cleaner, more efficient fusion reactors. The promise of reduced radioactive waste and higher energy conversion efficiencies makes He-3 a highly attractive alternative to D-T fusion.
However, the path to realizing this vision is fraught with challenges.
The Scarcity Problem: Where to Find Helium-3
The biggest hurdle facing Helium-3 fusion is its extreme scarcity on Earth. Unlike deuterium, which is abundant in seawater, and tritium, which can be bred from lithium, naturally occurring He-3 is exceptionally rare.
The Earth's atmosphere contains only trace amounts of He-3, and terrestrial sources are insufficient to support a large-scale fusion power industry.
Lunar Helium-3: A Potential Source?
The Moon, however, is believed to contain significant deposits of Helium-3 embedded in its regolith (surface soil). Over billions of years, the lunar surface has been bombarded by solar wind, which carries He-3 from the Sun.
This has led to considerable speculation about mining Helium-3 on the Moon and transporting it back to Earth for use in fusion reactors.
However, lunar He-3 mining faces numerous technical and economic challenges, including:
-
Low Concentrations: He-3 concentrations in lunar regolith are very low, requiring the processing of vast quantities of material to extract usable amounts.
-
Harsh Lunar Environment: The Moon's harsh environment, characterized by extreme temperatures, vacuum conditions, and radiation, poses significant challenges for mining operations.
-
High Transportation Costs: Transporting He-3 from the Moon to Earth would be extremely expensive and require significant advances in space transportation technology.
Technological Hurdles: Achieving D-He3 Fusion
Even if sufficient quantities of Helium-3 could be obtained, achieving efficient D-He3 fusion is technically challenging.
Compared to D-T fusion, the D-He3 reaction requires significantly higher plasma temperatures and confinement times. This places even greater demands on reactor design and plasma control technologies.
Cost Considerations: An Economic Balancing Act
The economic viability of Helium-3 fusion depends on a complex interplay of factors, including the cost of He-3 extraction and transportation, the efficiency of fusion reactors, and the market price of electricity.
Given the high costs associated with lunar mining and space transportation, He-3 fusion may only be economically feasible if fusion reactors can achieve extremely high performance levels and if the demand for clean energy drives up electricity prices significantly.
A Distant Prospect or a Future Possibility?
While the potential benefits of Helium-3 fusion are undeniable, the challenges associated with its scarcity, technical complexity, and economic viability are formidable.
Currently, Helium-3 fusion remains a distant prospect, with most fusion research focused on the more readily achievable D-T reaction.
However, continued advances in fusion technology, coupled with the growing demand for clean and sustainable energy sources, could potentially revive interest in Helium-3 as a futuristic fusion fuel. For now, it remains a tantalizing, albeit highly challenging, avenue for exploration in the quest for limitless fusion energy.
Helium-3 presents an enticing vision, yet its scarcity on Earth casts a long shadow. Finding practical and economically viable sources remains a Herculean task, even as research pushes forward to understand its potential. The reality is, for fusion to truly revolutionize our energy landscape, we must confront and overcome numerous obstacles that stand in the way of commercialization.
The Future of Fusion Chemistry: Path to Commercialization
Fusion energy, while promising, still faces significant hurdles before it can become a commercially viable energy source. These challenges span technological, economic, and regulatory domains. Overcoming these obstacles is crucial for realizing the dream of clean, abundant fusion power.
Navigating the Thorny Path: Hurdles to Commercialization
Several key challenges remain on the road to fusion commercialization:
-
Technological Maturity: Current fusion technologies, both magnetic and inertial confinement, are still in the experimental phase. Sustained, high-gain fusion reactions need to be consistently achieved and maintained. This requires advances in plasma control, materials science, and reactor design.
-
Materials Challenges: Fusion reactors operate under extreme conditions: intense heat, high pressures, and constant neutron bombardment. Developing materials that can withstand these conditions for extended periods is critical. New alloys and advanced composite materials are needed to ensure reactor longevity and reliability.
-
Fuel Availability: While deuterium is readily available from seawater, tritium is scarce and radioactive. The development of efficient tritium breeding technologies is essential for a self-sufficient fusion fuel cycle. Lithium blankets, used to breed tritium, must be optimized for efficiency and safety.
-
Economic Viability: The construction and operation of fusion power plants are incredibly expensive. Reducing costs through innovative engineering and manufacturing techniques is vital for making fusion competitive with other energy sources. Scalable and cost-effective reactor designs are needed to attract investment.
-
Regulatory Framework: Clear and consistent regulatory frameworks are needed to govern the licensing, construction, and operation of fusion power plants. These frameworks must address safety, environmental impact, and waste management.
A World Transformed: Long-Term Impact of Fusion Energy
If these hurdles can be overcome, the long-term impact of fusion energy on energy production and beyond could be profound:
-
Abundant Clean Energy: Fusion offers the potential for a virtually inexhaustible supply of clean energy. Deuterium, a primary fusion fuel, is readily available from seawater, ensuring a sustainable fuel source.
-
Reduced Greenhouse Gas Emissions: Fusion reactions do not produce greenhouse gases, making it a crucial tool in combating climate change. Widespread adoption of fusion power could significantly reduce global carbon emissions.
-
Energy Independence: Fusion could enable countries to achieve energy independence by relying on domestically available resources. This would reduce reliance on fossil fuel imports and enhance energy security.
-
Economic Growth: The development and deployment of fusion technologies could create new industries and jobs, stimulating economic growth. Investment in fusion research and development can drive innovation and create high-tech jobs.
-
Beyond Energy Production: Fusion technology could have applications beyond energy production, such as materials science, medicine, and space exploration. The high-energy plasmas generated in fusion reactors can be used for advanced materials processing and medical isotope production.
The Engine of Progress: Research, Innovation, and Collaboration
Continued research and innovation are crucial for shaping the future of fusion chemistry:
-
Fundamental Research: Basic research into plasma physics, materials science, and fusion reactions is essential for advancing our understanding of fusion processes. Funding for fundamental research is vital for laying the groundwork for future breakthroughs.
-
Technology Development: Developing and testing new fusion technologies, such as advanced reactor designs and fuel cycle systems, is critical for improving performance and reducing costs. Public-private partnerships can accelerate technology development and deployment.
-
International Collaboration: Fusion research is a global endeavor, requiring collaboration among scientists and engineers from around the world. International projects like ITER play a crucial role in advancing fusion science and technology.
-
Education and Training: Investing in education and training programs is essential for building a skilled workforce to support the development and deployment of fusion energy. Universities and research institutions must train the next generation of fusion scientists and engineers.
By addressing these challenges through sustained research, innovation, and collaboration, we can unlock the full potential of fusion chemistry and pave the way for a sustainable energy future.
Video: Fusion Chemistry: The Ultimate Guide You Need to Read
Frequently Asked Questions about Fusion Chemistry
Here are some common questions we receive about fusion chemistry and the processes involved.
What exactly is fusion chemistry?
Fusion chemistry, at its core, describes the chemical reactions and principles involved in nuclear fusion. It’s the study of how atoms combine at extremely high temperatures and pressures to form new, heavier elements, releasing tremendous energy. It's not chemistry in the traditional sense of bonding between atoms, but rather the manipulation of the nucleus itself.
Why is fusion chemistry so difficult to achieve?
Achieving controlled fusion chemistry is incredibly difficult due to the extreme conditions required. Overcoming the electrostatic repulsion between positively charged nuclei demands immense energy, typically achieved through very high temperatures and pressures, which are challenging to maintain and control in a stable manner.
What are the potential benefits of successful fusion chemistry?
Successful fusion chemistry holds the promise of a virtually limitless and clean energy source. Fusion reactions produce little to no greenhouse gases and utilize abundant fuels like deuterium and tritium, derived from seawater. This could revolutionize energy production and help combat climate change.
What role does plasma play in fusion chemistry?
Plasma is a crucial element in most fusion energy research. The extreme temperatures needed for fusion cause the fuel to exist in a plasma state – a superheated, ionized gas. This plasma must be carefully confined and controlled, often using strong magnetic fields, to prevent it from cooling and halting the fusion reaction. The stability of this plasma is critical for sustained fusion chemistry.