Unlock Carbon's Secrets: Valence Electrons Explained!
Understanding carbon valence electrons is foundational to grasping organic chemistry. The properties of diamond, a crystalline allotrope of carbon, are directly related to the configuration of these electrons. Linus Pauling's work on the nature of the chemical bond significantly advanced our understanding of how carbon valence electrons participate in covalent bonding. The concept also underpins the development of novel materials in laboratories like those at the Lawrence Berkeley National Laboratory, where researchers explore carbon-based nanostructures. Therefore, a solid understanding of carbon valence electrons is crucial for students and researchers alike seeking to explore the fascinating world of carbon compounds.
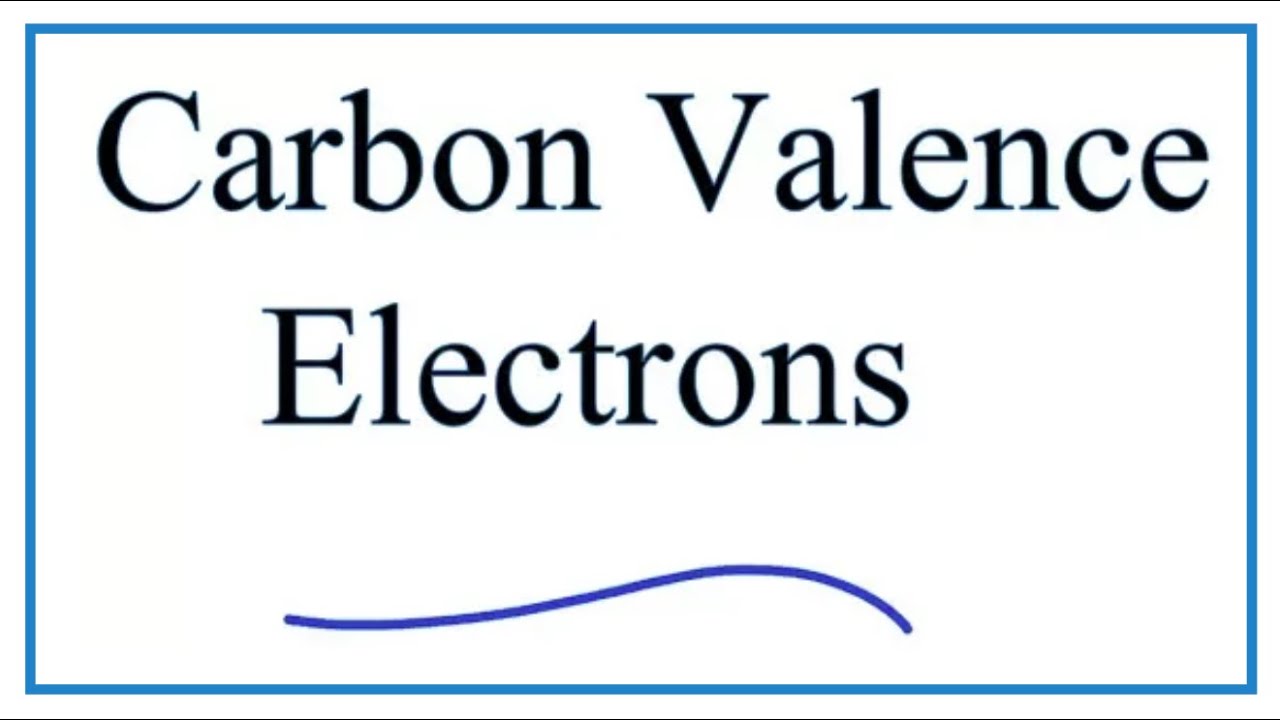
Image taken from the YouTube channel Wayne Breslyn (Dr. B.) , from the video titled Valence Electrons in Carbon (C) .
Carbon, the backbone of organic chemistry and life itself, owes its remarkable versatility to its valence electrons.
These outermost electrons dictate how carbon interacts with other atoms, forming the chemical bonds that create a vast and diverse array of molecules.
A solid grasp of valence electrons is crucial to understanding carbon's unique behavior.
Defining Valence Electrons and Their Role
Valence electrons are the electrons in the outermost electron shell of an atom.
These electrons are primarily responsible for determining the chemical properties of an element and how it participates in chemical bonding.
Atoms strive to achieve a stable electron configuration, often resembling that of a noble gas, which typically involves having eight valence electrons (the octet rule).
Atoms achieve this stability through the sharing or transfer of electrons, resulting in the formation of chemical bonds.
These bonds hold atoms together to form molecules.
Carbon's Unique Ability: Four Covalent Bonds
Carbon possesses a distinctive characteristic that sets it apart from many other elements: its ability to form four covalent bonds.
With four valence electrons, carbon needs four more electrons to complete its octet.
It readily achieves this by sharing electrons with other atoms, forming strong covalent bonds.
This tetravalency allows carbon to bond with a wide variety of elements, including hydrogen, oxygen, nitrogen, and even other carbon atoms.
This creates an almost limitless possibility of molecular structures.
This capacity is fundamental to the structural complexity and functional diversity observed in organic molecules.
Why Valence Electrons Matter in Organic Chemistry
Understanding carbon's valence electrons is paramount for comprehending its role in organic chemistry.
The arrangement and behavior of these electrons directly influence the structure, reactivity, and properties of organic molecules.
By understanding how carbon forms bonds, chemists can predict and explain the behavior of organic compounds.
Also, how they participate in chemical reactions.
This knowledge is essential for designing new molecules with specific properties.
It can be used for developing new materials, pharmaceuticals, and other essential products.
Understanding carbon's unique behavior hinges on grasping the fundamental role of valence electrons. Carbon's ability to form an extraordinary diversity of molecules is a direct consequence of these interactions. Now, let's shift our focus to the carbon atom itself, the very foundation upon which the vast and intricate structures of organic chemistry are built.
Carbon Atoms: The Building Blocks of Organic Molecules
The carbon atom stands as the cornerstone of organic chemistry, an element with properties uniquely suited to the creation of a dazzling array of molecules. Its electronic structure and its inherent drive to form stable bonds with other elements make it the central player in the drama of life itself.
The Electronic Structure of Carbon
The key to understanding carbon's behavior lies within its electronic structure.
Carbon has an atomic number of 6, meaning it possesses 6 protons and, in a neutral atom, 6 electrons. These electrons are arranged in specific energy levels, or electron shells, surrounding the nucleus.
The first shell, closest to the nucleus, can hold a maximum of two electrons.
The second shell can accommodate up to eight electrons.
Atomic Number and Electron Shells
The atomic number is the number of protons found in the nucleus of every atom of that element.
It defines the element's identity and determines its position on the periodic table.
Electrons occupy regions of space around the nucleus called electron shells or energy levels.
Each shell can hold a specific number of electrons.
The first shell holds a maximum of two electrons. The second shell can hold up to eight.
The arrangement of electrons in these shells dictates how an atom interacts with other atoms.
Carbon's Quest for an Octet
Carbon has two electrons in its first shell and four electrons in its second shell. This means it requires four more electrons to complete its octet – the stable configuration of eight electrons in the outermost shell, resembling that of a noble gas.
This is the driving force behind carbon's bonding behavior.
Carbon achieves this stability by sharing electrons with other atoms, forming covalent bonds.
This tendency to form four covalent bonds, known as tetravalency, is what allows carbon to create such a diverse range of molecular structures. The implications of this simple electronic structure are staggering.
From the simplest hydrocarbons to the most complex proteins and DNA, carbon's ability to link with itself and other elements allows for the construction of an endless variety of molecules, each with its unique properties and functions.
Understanding how carbon atoms are constructed provides a solid base for comprehending their function. Now, let's shift our gaze towards the architecture of carbon itself. This will reveal precisely how its electron configuration dictates its remarkable ability to bind with other elements, paving the way for the incredible variety of molecules we see in the organic world.
Electron Configuration and Carbon's Bonding Capacity
The diversity of organic molecules stems directly from carbon's unique electron configuration, which dictates its bonding capacity.
By understanding this arrangement, we can begin to unravel the secrets of carbon's versatility.
Deciphering Carbon's Electron Configuration
Carbon's electron configuration is represented as 1s22s22p2. This notation reveals how carbon's six electrons are distributed across its energy levels and orbitals.
The 1s orbital, closest to the nucleus, is filled with two electrons. The 2s orbital also contains two electrons.
Finally, the 2p orbitals hold the remaining two electrons.
The Dance of 2s and 2p Orbitals in Bonding
The 2s and 2p orbitals are crucial in understanding how carbon bonds with other atoms. These orbitals constitute carbon's valence shell, the outermost electron shell involved in chemical bonding.
The four electrons in these orbitals allow carbon to form up to four covalent bonds.
This tetravalency is a fundamental property that sets carbon apart.
These bonds can be single, double, or triple, leading to an immense diversity of molecular structures.
Hybridization: A Glimpse into Carbon's Bonding Prowess
While a full discussion of hybridization is beyond the scope of this section, it's important to briefly introduce the concept. Hybridization is the mixing of atomic orbitals to form new hybrid orbitals suitable for bonding.
Carbon readily undergoes hybridization, resulting in sp3, sp2, and sp hybridized orbitals.
Each type of hybridization leads to different molecular geometries and bonding characteristics. The concept of hybridization further explains carbon’s ability to form stable and diverse structures.
Chemical Bonding: Covalent Bonds and Carbon
Having explored the intricacies of carbon's electron configuration, we can now turn our attention to the bonds it forms. These bonds are the very glue that holds organic molecules together. Understanding their nature is critical to understanding the behavior of carbon and the compounds it creates.
Defining Covalent Bonds
Covalent bonds are the cornerstone of organic chemistry, distinguished by the sharing of electrons between atoms. Unlike ionic bonds, where electrons are transferred, covalent bonds involve a mutual attraction to the shared electrons, effectively linking the atoms.
This sharing allows atoms to achieve a more stable electron configuration. This fulfills the octet rule (or duet rule for hydrogen).
Carbon's Quest for a Stable Octet
Carbon, with its four valence electrons, has a strong tendency to form covalent bonds. By sharing electrons with other atoms, carbon can attain a full outer shell of eight electrons, mirroring the stable configuration of noble gases.
This drive for stability is the engine behind carbon's prolific bonding behavior. This allows it to form chains, rings, and complex three-dimensional structures.
Single, Double, and Triple Bonds: A Spectrum of Connectivity
Carbon's unique bonding capacity allows it to form single, double, and triple covalent bonds. This versatility significantly expands the structural diversity of organic molecules. Each type of bond represents a different degree of electron sharing and, consequently, exhibits distinct properties.
Single Bonds: The Foundation
A single bond consists of one shared pair of electrons between two atoms. Single bonds are the most common type of covalent bond.
They are relatively weak compared to double or triple bonds. They allow for free rotation around the bond axis. This flexibility contributes to the dynamic nature of molecules.
Double Bonds: A Step Up in Strength
A double bond involves the sharing of two pairs of electrons. This sharing creates a stronger and shorter bond than a single bond.
The presence of a double bond restricts rotation around the bond axis. This restriction introduces rigidity and influences molecular shape.
Triple Bonds: The Ultimate Connection
A triple bond, the strongest and shortest of the three, involves the sharing of three pairs of electrons.
Like double bonds, triple bonds completely restrict rotation around the bond axis. This leads to linear geometries around the involved atoms.
Bond Strength and Length: An Inverse Relationship
There's a clear inverse relationship between bond length and bond strength:
- Shorter bonds are stronger, requiring more energy to break.
- Longer bonds are weaker, requiring less energy to break.
This relationship arises from the increased electron density and attractive forces associated with multiple bonds.
Examples in Molecules
- Single Bond: Ethane (C2H6) features a single carbon-carbon bond.
- Double Bond: Ethene (C2H4), also known as ethylene, showcases a double carbon-carbon bond.
- Triple Bond: Ethyne (C2H2), commonly called acetylene, exhibits a triple carbon-carbon bond.
These examples illustrate how the type of bond influences the properties and reactivity of organic molecules.
The Octet Rule and Lewis Structures for Carbon Compounds
Having explored the nature of single, double, and triple bonds, it's time to understand how to represent these bonds visually and predict the stability of carbon compounds. This involves understanding the octet rule and the tool we use to visualize it: Lewis structures.
Understanding the Octet Rule
The octet rule is a fundamental principle in chemistry that dictates the tendency of atoms to achieve a stable electron configuration similar to that of noble gases. In most cases, this means having eight valence electrons in their outermost shell.
Atoms achieve this stability by gaining, losing, or, most importantly for carbon, sharing electrons through covalent bonds. Hydrogen, with only one electron shell, follows a duet rule, aiming for two electrons.
The octet rule is more of a guideline than a strict law. There are exceptions, particularly with elements beyond the second row of the periodic table. However, it remains a powerful tool for understanding and predicting the structure and stability of many organic molecules, particularly those containing carbon, nitrogen, oxygen, and hydrogen.
Drawing Lewis Structures for Carbon Compounds
Lewis structures are visual representations of molecules that show how atoms are connected and how valence electrons are distributed. They are essential for understanding bonding patterns and predicting molecular properties. Here's how to construct them, with examples tailored to carbon compounds:
-
Count the Total Number of Valence Electrons: Sum the valence electrons of all atoms in the molecule. For example, methane (CH4) has 4 (from carbon) + 4 1 (from hydrogen) = 8 valence electrons. Carbon dioxide (CO2) has 4 (from carbon) + 2 6 (from oxygen) = 16 valence electrons.
-
Draw the Skeletal Structure: Place the atoms in their likely arrangement. Carbon is typically the central atom because of its tetravalency. Connect the atoms with single bonds, representing shared electron pairs.
-
Distribute the Remaining Electrons as Lone Pairs: Start by placing electron pairs (dots) around the surrounding atoms to satisfy their octets (or duet for hydrogen).
-
Complete the Octet of the Central Atom: If the central atom (carbon) does not have an octet, form multiple bonds (double or triple) by sharing lone pairs from the surrounding atoms.
-
Check for Formal Charges (Optional): Formal charge can help determine the most stable Lewis structure when multiple possibilities exist. However, this is a more advanced concept.
Example 1: Methane (CH4)
- Total valence electrons: 8
- Carbon as the central atom bonded to four hydrogen atoms.
- Each single bond represents two shared electrons. Carbon has an octet, and each hydrogen has a duet.
The Lewis structure for methane consists of a central carbon atom with four single bonds to four hydrogen atoms, and no lone pairs.
Example 2: Carbon Dioxide (CO2)
- Total valence electrons: 16
- Carbon as the central atom, bonded to two oxygen atoms.
- Connect carbon to each oxygen with a single bond.
This uses 4 electrons (2 bonds x 2 electrons/bond). We're left with 12 electrons. Place 3 lone pairs on each oxygen atom. This uses all 12 remaining electrons. The carbon atom only has 4 electrons around it (2 single bonds). To give carbon an octet, form a double bond between the carbon and each oxygen. The final Lewis structure for carbon dioxide consists of a carbon atom double-bonded to each of the two oxygen atoms. Each oxygen atom also has two lone pairs.
Bonding and Non-Bonding Electrons
In a Lewis structure, electrons are classified as either bonding or non-bonding.
-
Bonding electrons are those that are shared between atoms in a covalent bond. Each single bond represents two bonding electrons, a double bond represents four, and a triple bond represents six.
-
Non-bonding electrons, also known as lone pairs, are valence electrons that are not involved in bonding. They reside on a single atom.
For example, in methane (CH4), all eight valence electrons are bonding electrons, forming four single bonds. In carbon dioxide (CO2), there are eight bonding electrons (four from each double bond) and eight non-bonding electrons (four lone pairs, two on each oxygen).
Understanding the distribution of bonding and non-bonding electrons is crucial for predicting molecular shape, polarity, and reactivity. These factors ultimately influence the physical and chemical properties of organic compounds.
Hybridization: sp3, sp2, and sp Orbitals
Having established the importance of Lewis structures in visualizing bonding patterns, it's crucial to understand the underlying theory that explains why carbon forms bonds the way it does. This leads us to the concept of hybridization, a cornerstone of understanding molecular geometry and bonding in organic chemistry.
Defining Orbital Hybridization
Orbital hybridization is the concept of mixing atomic orbitals to form new hybrid orbitals suitable for the pairing of electrons to form chemical bonds in valence bond theory.
Think of it as a mathematical procedure that combines standard atomic orbitals to generate new, hybridized orbitals that are lower in energy and allow for stronger, more stable covalent bonds.
Hybridization is crucial because it allows carbon to form the required number of bonds with the correct geometry for maximum stability. It is not something that occurs in an isolated atom. Rather, it is a model to explain molecular geometries.
sp3 Hybridized Orbitals: The Tetrahedral Carbon
Formation and Characteristics of sp3 Orbitals
When carbon forms four single bonds, as in methane (CH4), it undergoes sp3 hybridization. In this process, one 2s orbital and three 2p orbitals mix to form four equivalent sp3 hybrid orbitals.
Each sp3 orbital has 25% s character and 75% p character, and they are oriented in space to minimize electron repulsion.
Tetrahedral Geometry and Methane (CH4)
The most important consequence of sp3 hybridization is the resulting tetrahedral geometry. The four sp3 orbitals point towards the corners of a tetrahedron, with bond angles of approximately 109.5 degrees.
This geometry is exemplified by methane (CH4), where the central carbon atom is sp3 hybridized and bonded to four hydrogen atoms, creating a perfectly tetrahedral molecule.
This tetrahedral shape is fundamental to understanding the three-dimensional structure of many organic molecules.
sp2 Hybridized Orbitals: The Trigonal Planar Carbon
Formation and Characteristics of sp2 Orbitals
When carbon forms one double bond and two single bonds, it undergoes sp2 hybridization.
In this process, one 2s orbital and two 2p orbitals mix to form three equivalent sp2 hybrid orbitals, leaving one unhybridized p orbital.
Each sp2 orbital has 33.3% s character and 66.7% p character.
Trigonal Planar Geometry and Ethene (C2H4)
The three sp2 orbitals are arranged in a trigonal planar geometry, with bond angles of approximately 120 degrees. The unhybridized p orbital is perpendicular to this plane.
Ethene (C2H4), also known as ethylene, is a classic example of an sp2 hybridized molecule. Each carbon atom is sp2 hybridized and forms a sigma (σ) bond with two hydrogen atoms and another carbon atom.
The remaining unhybridized p orbitals on each carbon atom overlap to form a pi (π) bond, resulting in the carbon-carbon double bond.
The planar structure and the presence of a pi bond are crucial to the reactivity of alkenes like ethene.
sp Hybridized Orbitals: The Linear Carbon
Formation and Characteristics of sp Orbitals
When carbon forms one triple bond and one single bond, or two double bonds, it undergoes sp hybridization. In this process, one 2s orbital and one 2p orbital mix to form two equivalent sp hybrid orbitals, leaving two unhybridized p orbitals.
Each sp orbital has 50% s character and 50% p character.
Linear Geometry and Ethyne (C2H2)
The two sp orbitals are arranged in a linear geometry, with a bond angle of 180 degrees. The two unhybridized p orbitals are perpendicular to each other and to the axis of the sp orbitals.
Ethyne (C2H2), also known as acetylene, is a prime example of an sp hybridized molecule. Each carbon atom is sp hybridized and forms a sigma (σ) bond with one hydrogen atom and the other carbon atom.
The two unhybridized p orbitals on each carbon atom overlap to form two pi (π) bonds, resulting in the carbon-carbon triple bond.
The linear structure and the presence of two pi bonds make alkynes like ethyne highly reactive. The high s-character leads to stronger bonds.
Having explored how sp3 hybridization leads to the formation of four equivalent bonds oriented in space, it's crucial to understand the ramifications of this arrangement. The resulting tetrahedral geometry isn't just a spatial curiosity; it's a fundamental determinant of molecular shape and reactivity, and it underpins much of the diversity observed in organic compounds.
Tetrahedral Geometry: Carbon's 3D Structure
The tetrahedral geometry around a carbon atom profoundly impacts the three-dimensional structure of molecules.
Instead of existing in a flat, two-dimensional plane, molecules with tetrahedral carbon centers adopt a three-dimensional arrangement. This seemingly simple shift has far-reaching implications for how molecules interact with each other and their environment.
The Foundation of Molecular Shape
Imagine methane (CH4), the simplest alkane. The central carbon atom is sp3 hybridized, resulting in four sigma bonds radiating outwards towards the hydrogen atoms.
These bonds repel each other, settling into a configuration where they are as far apart as possible – the corners of a tetrahedron.
This arrangement results in bond angles of approximately 109.5 degrees, which defines the tetrahedral shape.
Understanding the geometry is crucial because molecular shape dictates many properties, including melting point, boiling point, and reactivity.
Tetrahedral Geometry and Molecular Diversity
The tetrahedral geometry of carbon is a critical factor in the vast diversity of organic compounds.
Because carbon can form four bonds, it can act as a branching point in a molecule.
This branching capability, combined with the specific bond angles enforced by the tetrahedral arrangement, allows for the creation of complex and varied molecular architectures.
Isomers, molecules with the same chemical formula but different structural arrangements, arise directly from this three-dimensionality. The subtle changes in spatial arrangement can dramatically alter a molecule's properties and function.
Influence on Molecular Interactions
The tetrahedral geometry of carbon plays a significant role in determining how molecules interact with each other through intermolecular forces.
Molecular shape influences how effectively molecules can pack together, affecting the strength of van der Waals forces.
Furthermore, the polarity of individual bonds, in conjunction with the overall molecular shape, determines the presence and strength of dipole-dipole interactions.
Consider how tetrahedral carbon centers contribute to the folding and function of proteins, where precise three-dimensional structures are essential for biological activity.
The shape of enzyme active sites, for example, is directly dependent on the geometry of the carbon atoms within the amino acid residues.
These examples highlight that carbon's tetrahedral geometry is not merely a structural detail but an active participant in determining a molecule's interactions and behavior.
Organic Chemistry: Unveiling the Realm of Carbon Compounds
Having considered the profound influence of tetrahedral geometry on molecular architecture, it's natural to transition into the broader context of organic chemistry, the discipline dedicated entirely to the study of carbon-based molecules. Understanding carbon's unique bonding capabilities is not merely an academic exercise; it's the cornerstone upon which the entire edifice of organic chemistry is built.
Defining the Scope of Organic Chemistry
Organic chemistry, at its core, is the study of compounds that contain carbon. This vast field encompasses a staggering array of molecules, from the simplest hydrocarbons to complex biomolecules like proteins and DNA.
It's important to note that not all carbon-containing compounds are considered organic. For instance, carbon dioxide (CO2), carbonates, and carbides are typically classified as inorganic compounds.
The distinction arises from historical reasons and the nature of the chemical bonds involved. Organic compounds generally feature carbon-carbon and carbon-hydrogen bonds, whereas inorganic carbon compounds often involve ionic or metallic bonding.
The Pivotal Role of Carbon's Valence Electrons
The exceptional diversity and complexity of organic molecules are directly attributable to carbon's unique electronic structure. With four valence electrons, carbon can form four covalent bonds, allowing it to create stable linkages with itself and a variety of other elements, including hydrogen, oxygen, nitrogen, and halogens.
This tetravalency enables carbon to form long chains, branched structures, and cyclic compounds, providing the structural framework for an enormous range of molecular architectures.
Furthermore, carbon's ability to form single, double, and triple bonds allows for even greater structural variation and influences the reactivity of organic molecules. The strength and length of these bonds directly impact molecular stability and the types of chemical reactions a molecule can undergo.
Functional Groups: Modifying Molecular Behavior
While carbon-carbon and carbon-hydrogen bonds form the backbone of most organic molecules, the presence of functional groups attached to this backbone dramatically alters their chemical and physical properties.
Functional groups are specific arrangements of atoms within a molecule that exhibit characteristic reactivity. Common examples include:
-
Hydroxyl groups (-OH), found in alcohols, which increase water solubility and participate in hydrogen bonding.
-
Carbonyl groups (C=O), present in aldehydes and ketones, which are highly reactive and involved in numerous chemical transformations.
-
Carboxyl groups (-COOH), characteristic of carboxylic acids, which exhibit acidic properties and participate in esterification reactions.
-
Amino groups (-NH2), found in amines, which act as bases and play a crucial role in the formation of amides and peptides.
The identity and position of these functional groups within a molecule dictate its reactivity, polarity, and interactions with other molecules. By understanding the properties of common functional groups, chemists can predict and control the behavior of organic compounds.
Carbon Allotropes: A Tale of Many Forms
Carbon, the cornerstone of organic chemistry, exhibits a remarkable ability to exist in various forms known as allotropes. These allotropes, including diamond, graphite, fullerenes, and graphene, demonstrate the profound impact of atomic arrangement and bonding on macroscopic properties. The same element, simply organized in different ways, can yield materials with drastically different characteristics.
Diamond: A Crystalline Fortress
Diamond, renowned for its exceptional hardness and brilliance, possesses a crystal structure where each carbon atom is tetrahedrally bonded to four other carbon atoms. This three-dimensional network of strong covalent bonds extends throughout the entire crystal, creating an incredibly rigid and resistant material.
The tight, interlocking structure explains diamond's unparalleled hardness, making it ideal for cutting tools and abrasives.
Furthermore, the uniform bonding and lack of free electrons contribute to diamond's excellent thermal conductivity and electrical insulation.
Its high refractive index also gives it the sparkle prized in jewelry.
Graphite: Slippery Layers of Strength
In stark contrast to diamond, graphite exhibits a layered structure. Each layer, known as graphene, consists of carbon atoms arranged in a hexagonal lattice. Within each layer, carbon atoms are strongly bonded to three other carbon atoms via sp2 hybridization.
However, the layers themselves are held together by weak van der Waals forces. This weak interlayer bonding allows the layers to easily slide past one another.
This explains graphite's softness and its utility as a lubricant.
The delocalized electrons within the graphene layers also make graphite electrically conductive. This property is exploited in electrodes, batteries, and other electronic applications.
Fullerenes: Spherical Elegance
Fullerenes, discovered more recently, represent a class of carbon allotropes with closed-cage structures. The most famous fullerene, buckminsterfullerene (C60), resembles a soccer ball, consisting of 60 carbon atoms arranged in a spherical structure with pentagonal and hexagonal faces.
Fullerenes exhibit unique properties, including the ability to encapsulate other atoms or molecules within their cages.
This has spurred research into their potential applications in drug delivery, nanotechnology, and materials science.
Their discovery opened up an entirely new avenue in carbon material research.
Graphene: The Wonder Material
Graphene, a single layer of carbon atoms arranged in a hexagonal lattice, is essentially a single sheet of graphite. It is remarkably strong, lightweight, and flexible.
Graphene possesses exceptional electrical and thermal conductivity, surpassing many other known materials.
Its high surface area and unique electronic properties make it a promising candidate for applications in electronics, energy storage, composites, and sensors.
Graphene is actively investigated for use in next-generation electronic devices and high-performance materials. Its discovery ignited an explosion of research in two-dimensional materials.
Video: Unlock Carbon's Secrets: Valence Electrons Explained!
FAQs: Carbon's Valence Electrons Explained
This FAQ section provides answers to common questions about carbon and its valence electrons. We aim to clarify key concepts from the article, making it easier to understand how carbon forms bonds.
Why are valence electrons so important for carbon?
Valence electrons are the outermost electrons of an atom. Carbon has four valence electrons, and these are the key to how carbon interacts with other atoms. Because carbon valence electrons determine the type and number of chemical bonds carbon can form, they are the reason carbon is so versatile.
How does carbon's number of valence electrons relate to the number of bonds it can make?
Carbon has four valence electrons, meaning it needs four more electrons to achieve a stable octet. This need drives carbon to form four covalent bonds. These four bonds can be single, double, or triple bonds, as long as the total number of bonds is four.
Can carbon share more than four valence electrons?
No, carbon only has four valence electrons to share. Each bond it forms involves sharing one or more of these valence electrons with another atom. It can't share more than the four it initially possesses as carbon valence electrons.
What are some examples of how carbon uses its valence electrons to bond?
Carbon can bond with four hydrogen atoms to form methane (CH4), with each hydrogen sharing one electron. It can also bond with two oxygen atoms in carbon dioxide (CO2), forming two double bonds where each oxygen shares two electrons. Carbon's ability to use its carbon valence electrons in different configurations makes it essential for organic chemistry.