Sun Spectral Type: A Beginner's Guide
The Morgan-Keenan (MK) system classifies stars, including our own, based on their spectral characteristics, influencing parameters like temperature and luminosity which astronomers analyze to determine a star's sun spectral type. A star's position on the Hertzsprung-Russell diagram correlates directly with its sun spectral type, providing insights into its evolutionary stage. The National Aeronautics and Space Administration (NASA) uses spectral analysis to study distant stars, comparing their properties to our Sun, an example of a G-type main-sequence star, aiding in the search for habitable exoplanets.
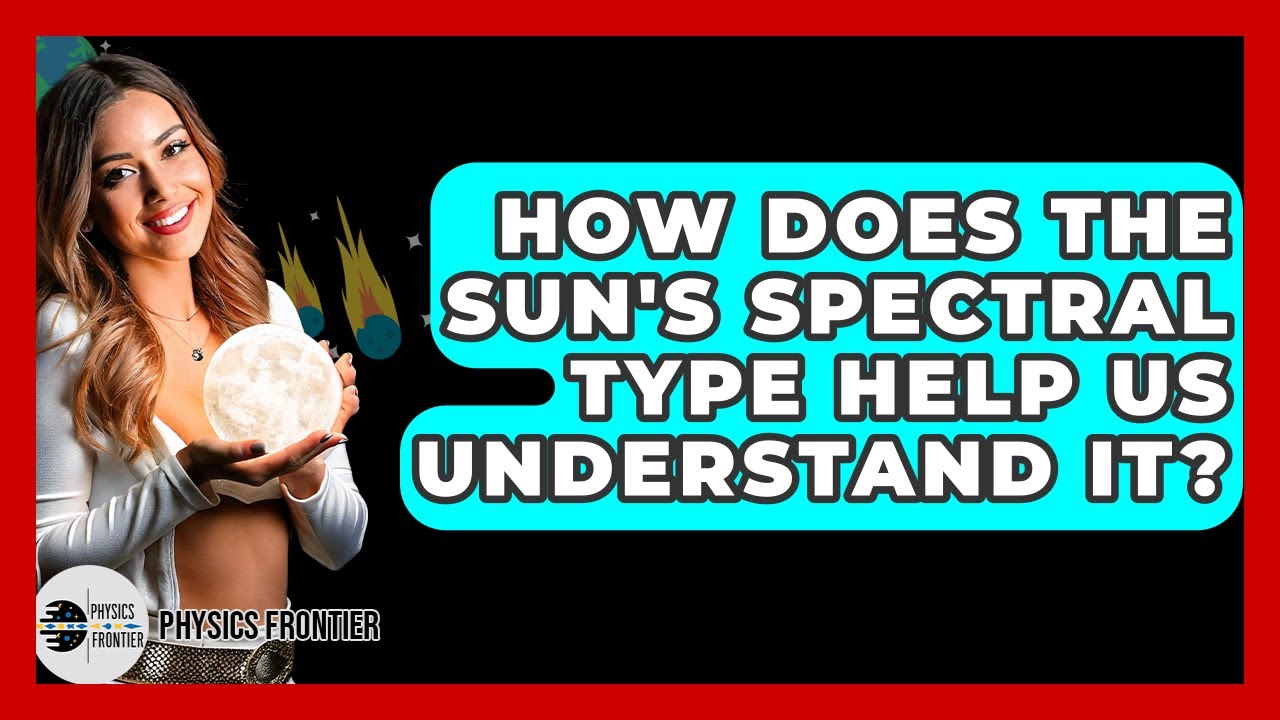
Image taken from the YouTube channel Physics Frontier , from the video titled How Does The Sun's Spectral Type Help Us Understand It? - Physics Frontier .
Stellar classification stands as a cornerstone of modern astrophysics, a meticulous process by which we categorize the vast population of stars based on their intrinsic properties. This classification, far from being a mere exercise in taxonomy, unlocks profound insights into the life cycles, compositions, and ultimate destinies of these celestial objects.
The Importance of Stellar Classification
At its core, stellar classification allows astronomers to organize the seemingly chaotic diversity of stars into manageable groups. By examining a star's spectrum – the unique fingerprint of light it emits – we can infer a wealth of information. This includes its temperature, luminosity, chemical composition, and even its age.
The Harvard System: A Foundation for Understanding
The Harvard Spectral Classification system is arguably the most widely used and recognized framework for classifying stars. This system arranges stars into spectral classes denoted by the letters O, B, A, F, G, K, and M, with each class further subdivided numerically. These letters, seemingly arbitrary, actually represent a sequence of decreasing stellar surface temperature.
Each spectral class exhibits distinct spectral features, primarily absorption lines, which reveal the elements present in the star's atmosphere and their ionization states. This classification is not merely descriptive; it's deeply rooted in the physics of stellar atmospheres and the processes that govern their light emission.
The Hertzsprung-Russell Diagram: Visualizing Stellar Populations
Complementing the Harvard system is the Hertzsprung-Russell (HR) Diagram, a powerful tool that plots stars according to their luminosity and spectral type (or temperature). The HR Diagram reveals striking patterns in the distribution of stars, with the majority falling along a diagonal band known as the Main Sequence.
This sequence represents stars that are fusing hydrogen into helium in their cores, the longest and most stable phase of stellar evolution. The HR Diagram also showcases other stellar populations, such as giants, supergiants, and white dwarfs, each representing distinct stages in the stellar life cycle.
Unlocking Stellar Evolution and Characteristics
By combining stellar classification with the HR Diagram, astronomers gain a comprehensive understanding of stellar evolution. The position of a star on the HR Diagram provides clues to its age, mass, and evolutionary history. As stars age, they move off the Main Sequence, undergoing dramatic changes in their size, luminosity, and temperature.
Through stellar classification, we can track these evolutionary paths and piece together the life stories of individual stars and entire stellar populations. Ultimately, these tools provide invaluable insight into the processes that shape the cosmos, from the formation of elements within stellar cores to the eventual fate of stars as white dwarfs, neutron stars, or black holes. The universe is a vast, complex place, and stellar classification provides a powerful framework for making sense of it all.
A Historical Journey Through Stellar Classification
Stellar classification stands as a cornerstone of modern astrophysics, a meticulous process by which we categorize the vast population of stars based on their intrinsic properties. This classification, far from being a mere exercise in taxonomy, unlocks profound insights into the life cycles, compositions, and ultimate destinies of these celestial bodies. The journey to our current understanding is a fascinating story of dedicated individuals and groundbreaking discoveries.
Early Efforts at the Harvard College Observatory
The genesis of modern stellar classification can be traced back to the Harvard College Observatory in the late 19th century. Driven by the desire to systematically catalog the ever-growing number of observed stars, the observatory embarked on ambitious projects to capture and analyze stellar spectra. These early efforts laid the groundwork for more sophisticated techniques.
Pickering's Vision: Large-Scale Spectral Surveys
Edward C. Pickering, the director of the Harvard College Observatory, played a pivotal role in initiating large-scale spectral surveys. He recognized the potential of photographic plates for capturing and preserving stellar spectra, allowing for detailed analysis and comparison. Pickering's vision transformed stellar astronomy from a qualitative field to a quantitative science.
The "Harvard Computers": Unsung Heroines of Astronomy
The meticulous task of analyzing the thousands of photographic plates fell to a dedicated team of women, often referred to as the "Harvard Computers." These unsung heroines, including Williamina Fleming, meticulously examined each spectrum, noting subtle differences and identifying recurring patterns. Fleming, in particular, is credited with classifying thousands of stars and discovering numerous novae.
Williamina Fleming: A Pioneer in Spectral Analysis
Fleming's work went far beyond simple data entry. She developed a system for classifying stars based on their hydrogen lines, initially using a system where stars were classified from A to Q, based on the strength of their Hydrogen spectral lines. Her contributions were essential to the early development of stellar classification.
Annie Jump Cannon and the OBAFGKM Sequence
Annie Jump Cannon stands as one of the most influential figures in the history of stellar classification. Building upon the work of her predecessors, Cannon refined the Harvard Spectral Classification system, eventually arriving at the familiar OBAFGKM sequence.
Cannon's insight was to realize that the spectral classes related to temperature. This was not obvious from the initial classification scheme.
This sequence, ordered by decreasing temperature, remains the standard for classifying stars today. Cannon personally classified hundreds of thousands of stars, leaving an enduring legacy in the field of astronomy.
The Hertzsprung-Russell Diagram: Connecting Spectra and Luminosity
Independently, Ejnar Hertzsprung and Henry Norris Russell developed a revolutionary tool for understanding stellar properties: the Hertzsprung-Russell (HR) Diagram. This diagram plots stars based on their spectral type (or temperature) and luminosity.
By correlating these two fundamental properties, the HR Diagram revealed distinct groupings of stars. The HR Diagram unveiled the relationship between a star's spectral type and its absolute magnitude, revealing the main sequence. It became an invaluable tool for studying stellar evolution. Its impact on our understanding of stellar evolution and the cosmos is immeasurable.
Decoding Stellar Spectra: The Harvard Spectral Classification System
Following the foundational groundwork laid by early astronomical pioneers, the establishment of a standardized system for classifying stars became paramount. The Harvard Spectral Classification system emerged as the definitive framework. It offered a structured method for categorizing stars based on their spectral characteristics. This section will dissect the intricacies of this system, shedding light on its underlying principles and its power in revealing the nature of stars.
The OBAFGKM Sequence: A Temperature-Based Ordering
The cornerstone of the Harvard Spectral Classification system is the OBAFGKM sequence. It is a sequence organizing stars by decreasing surface temperature. It progresses from the hottest, most massive stars (O type) to the coolest, least massive stars (M type). Each letter corresponds to a distinct range of temperatures and spectral features.
The mnemonic "Oh, Be A Fine Girl/Guy, Kiss Me" is a common tool for remembering the order of these spectral classes. While seemingly simple, this sequence is rooted in the fundamental physics governing stellar atmospheres and the behavior of elements at varying temperatures.
Spectral Class Deep Dive: Characteristics and Key Features
Each spectral class exhibits unique spectral features due to differences in temperature and atmospheric composition.
-
O Stars: These are the hottest and most luminous stars, with surface temperatures exceeding 30,000 K. Their spectra are dominated by absorption lines of ionized helium and strong ultraviolet emission. They are relatively rare and short-lived.
-
B Stars: B-type stars are also hot, with temperatures ranging from 10,000 to 30,000 K. Neutral helium lines are prominent in their spectra, along with hydrogen lines. They are bluer in color compared to later-type stars.
-
A Stars: A stars have surface temperatures between 7,500 and 10,000 K. They exhibit strong hydrogen absorption lines, particularly the Balmer series. The iconic Sirius is a prime example.
-
F Stars: F-type stars are slightly cooler, with temperatures ranging from 6,000 to 7,500 K. Their spectra show weaker hydrogen lines and the emergence of metallic lines, such as ionized calcium.
-
G Stars: These stars, including our Sun, have surface temperatures between 5,200 and 6,000 K. Their spectra are characterized by strong absorption lines of ionized calcium and other metals. G stars are yellowish in color.
-
K Stars: K-type stars are cooler still, with temperatures ranging from 3,700 to 5,200 K. Their spectra exhibit strong metallic lines and molecular bands. They are orangeish in color.
-
M Stars: M stars are the coolest and most common type of stars, with surface temperatures below 3,700 K. Their spectra are dominated by molecular bands of titanium oxide (TiO) and other molecules. They are reddish in color.
Effective Temperature: The Driving Force
Effective Temperature is a crucial parameter in determining a star's spectral type. It is defined as the temperature of a blackbody that would emit the same total amount of electromagnetic radiation as the star. In essence, it represents the star's surface temperature.
The OBAFGKM sequence is fundamentally a temperature sequence. Stars are ordered based on their effective temperatures. This is because temperature dictates which elements can exist in specific ionization states. It also determines the strength of their corresponding absorption lines.
Numerical Subdivisions: Fine-Tuning the Classification
To further refine the classification, each spectral class is subdivided using numerical suffixes from 0 to 9. A0 represents the hottest stars within the A class, while A9 represents the coolest.
For example, an A5 star is cooler than an A0 star. But it is hotter than an F0 star. These numerical subdivisions allow for a more precise categorization. They provide a finer level of detail within each spectral class.
Beyond Spectral Type: Introducing Luminosity Classes
Following the foundational groundwork laid by early astronomical pioneers, the establishment of a standardized system for classifying stars became paramount. The Harvard Spectral Classification system emerged as the definitive framework. It offered a structured method for categorizing stars based on their spectral characteristics. However, spectral type alone is insufficient to fully describe a star's nature. The subtle differences in luminosity among stars of the same spectral type necessitate a further level of classification: luminosity classes.
Unveiling Luminosity Classes: A Deeper Dive
Luminosity classes provide a means to differentiate stars with similar surface temperatures but varying intrinsic brightness. These classes, designated by Roman numerals I through V, correlate directly with a star's size and luminosity. Understanding these classes allows astronomers to determine a star’s evolutionary state and refine distance measurements.
The Five Principal Luminosity Classes
Each luminosity class corresponds to a distinct region on the Hertzsprung-Russell (HR) diagram, reflecting different stages in a star's life cycle.
-
Class I - Supergiants: These are the most luminous and massive stars, having evolved off the main sequence. They are subdivided into:
- Ia: Luminous Supergiants
- Ib: Less Luminous Supergiants
These stars are rare and represent the final stages of massive stars. Examples include Betelgeuse and Rigel.
-
Class II - Bright Giants: Less luminous than supergiants, these stars are still considerably larger and brighter than main sequence stars. These represent a transition stage as stars evolve from the main sequence towards the giant branch.
-
Class III - Giants: These are evolved stars that have exhausted their core hydrogen fuel and have expanded in size. Many prominent stars in the night sky are giants. Examples include Arcturus and Aldebaran.
-
Class IV - Subgiants: This class represents stars that are in the process of evolving from the main sequence to the giant branch. They are slightly more luminous than main sequence stars of the same spectral type. These stars are transitioning and possess characteristics that place them between main sequence and giant stars.
-
Class V - Main Sequence Stars (Dwarfs): These are the most common type of stars, fusing hydrogen into helium in their cores. Our Sun is a main sequence star. This class represents stars in the longest and most stable phase of their lives.
Luminosity Classes on the HR Diagram
The Hertzsprung-Russell diagram visually represents the relationship between a star's luminosity and its temperature or spectral type. Stars of different luminosity classes occupy distinct regions.
-
Main sequence stars (Class V) form a diagonal band running from the upper left (hot, luminous stars) to the lower right (cool, faint stars).
-
Giants (Class III) and Supergiants (Class I) are located above the main sequence, reflecting their higher luminosity.
-
The position of a star on the HR diagram, combined with its spectral type, allows astronomers to estimate its distance using a technique called spectroscopic parallax.
Decoding Subtle Spectral Signatures
Determining a star's luminosity class requires careful analysis of its spectrum. While spectral type is primarily determined by temperature-sensitive absorption lines, luminosity class is inferred from subtle variations in line widths and intensities.
Pressure Broadening: A Key Indicator
- In denser stellar atmospheres (like those of main sequence stars), the increased pressure causes spectral lines to broaden due to collisions between atoms.* This effect, known as pressure broadening, is less pronounced in the extended, low-density atmospheres of giants and supergiants.
Ionization Levels and Line Intensities
The ionization levels and intensities of certain spectral lines are also sensitive to luminosity. In higher-luminosity stars, the lower atmospheric density leads to a different ionization balance. This subtly alters the strength of particular absorption lines. By meticulously measuring these spectral features, astronomers can accurately classify stars based on their luminosity.
Unlocking Stellar Secrets: Spectral Features and Analysis
Following the foundational groundwork laid by early astronomical pioneers, the establishment of a standardized system for classifying stars became paramount. The Harvard Spectral Classification system emerged as the definitive framework. It offered a structured method for categorizing stars based...
...not just on their apparent brightness, but on the intricate patterns embedded within their spectra. These patterns, specifically absorption lines, hold the key to unlocking a star's fundamental properties, from its elemental composition to its temperature and density.
The Significance of Absorption Lines
Absorption lines appear as dark lines in a star's continuous spectrum. They arise when photons of specific wavelengths are absorbed by atoms or ions in the star's atmosphere.
Each element has a unique set of energy levels, meaning that it absorbs light only at specific wavelengths. This creates a distinctive "fingerprint" in the spectrum, allowing astronomers to identify the elements present in the star's atmosphere.
The intensity of these absorption lines also provides crucial information about the physical conditions within the star. Temperature and density affect the degree to which elements are ionized or excited, altering the strength of their absorption lines.
Spectrographs: Capturing Stellar Light
The instrument used to capture and analyze stellar spectra is the spectrograph. This device takes the light gathered by a telescope and spreads it out into its component wavelengths, creating a spectrum.
Modern spectrographs often use diffraction gratings or prisms to disperse the light, and electronic detectors (such as CCDs) to record the spectrum. The resulting data can then be analyzed using specialized software.
The spectral data acquisition process typically involves several steps:
-
Calibration: Obtaining calibration spectra to correct for instrumental effects.
-
Wavelength Calibration: Establishing the precise relationship between pixel position and wavelength.
-
Flux Calibration: Converting the raw data into a measure of the star's energy output at each wavelength.
Elemental Abundances and Spectral Analysis
The relationship between spectral lines and elemental abundances is complex. The strength of an absorption line depends not only on the abundance of the element, but also on the temperature, density, and pressure within the star's atmosphere.
Detailed spectral analysis involves comparing observed spectra with theoretical models. These models, calculated using sophisticated computer codes, predict the expected spectrum for a given set of stellar parameters.
By comparing the observed and predicted spectra, astronomers can estimate the star's elemental abundances, temperature, density, and surface gravity. This process requires careful consideration of various factors, including:
-
Line Broadening: The broadening of spectral lines due to thermal motion, pressure, and rotation.
-
Non-LTE Effects: Deviations from local thermodynamic equilibrium, which can affect the ionization and excitation balance of elements.
-
Model Atmospheres: The accuracy of the stellar atmosphere models used in the analysis.
Despite these challenges, spectral analysis remains a powerful tool for unraveling the secrets of stars. By carefully studying the patterns of absorption lines, astronomers can gain insights into the composition, structure, and evolution of these distant celestial objects.
The Hertzsprung-Russell Diagram: A Stellar Roadmap
Following the foundational groundwork laid by early astronomical pioneers, the establishment of a standardized system for classifying stars became paramount. The Harvard Spectral Classification system emerged as the definitive framework. It offered a structured method for categorizing stars. However, a crucial piece was still needed to fully unlock the secrets encoded within stellar spectra. That piece was the Hertzsprung-Russell Diagram. The HR Diagram is a potent visual tool that would forever transform our understanding of stellar evolution and the very nature of stars.
Deciphering the HR Diagram's Structure
The Hertzsprung-Russell Diagram, often abbreviated as the HR Diagram, serves as a fundamental tool in astrophysics. It plots stars based on their luminosity against their temperature (or spectral type).
Typically, luminosity is displayed on the vertical axis, increasing upwards.
Temperature or spectral type resides on the horizontal axis, with temperature decreasing from left to right (O-type stars on the left, M-type stars on the right). The genius of this diagram lies in its ability to reveal patterns and relationships among stellar properties.
The Main Sequence: Where Stars Spend Their Prime
The most prominent feature of the HR Diagram is the Main Sequence. This diagonal band stretches from the upper left (hot, luminous stars) to the lower right (cool, faint stars).
It represents the vast majority of stars in our galaxy, including our own Sun. Stars on the Main Sequence are in the stable phase of their lives. They are undergoing core hydrogen fusion, converting hydrogen into helium. A star's position on the Main Sequence is primarily determined by its mass. More massive stars are hotter and more luminous, residing at the upper end, while less massive stars are cooler and fainter, found at the lower end.
The time a star spends on the Main Sequence is also mass-dependent. More massive stars burn through their fuel at a faster rate, resulting in shorter lifespans.
Beyond the Main Sequence: Giants, Supergiants, and White Dwarfs
While the Main Sequence dominates the HR Diagram, it's not the only stellar neighborhood. As stars exhaust the hydrogen fuel in their cores, they evolve off the Main Sequence and move into different regions of the diagram.
Giants and Supergiants are located above the Main Sequence. These are evolved stars that have expanded in size and increased in luminosity. Giants are typically less massive than Supergiants.
Giants have evolved off the main sequence and have begun fusing helium in their cores. Supergiants represent the evolved products of the most massive stars. They can undergo multiple stages of fusion, synthesizing heavier elements.
At the opposite end of the HR Diagram, in the lower left corner, reside the White Dwarfs.
These are the remnants of low- to intermediate-mass stars that have shed their outer layers. They are extremely dense, hot, and faint. White dwarfs are no longer undergoing nuclear fusion and are slowly cooling down over billions of years. Their position on the HR Diagram reflects their unique characteristics.
The distribution of stars in these different regions provides crucial insights into stellar evolution and the life cycles of stars. The HR Diagram serves as an indispensable tool for unraveling the complexities of the stellar population.
Applications of the HR Diagram: Measuring the Cosmos
Following the foundational groundwork laid by early astronomical pioneers, the establishment of a standardized system for classifying stars became paramount. The Harvard Spectral Classification system emerged as the definitive framework. It offered a structured method for categorizing stars. However, the true power of stellar classification is realized when combined with the Hertzsprung-Russell (HR) Diagram, transforming it from a mere sorting tool into a powerful analytical instrument for measuring cosmic distances, unraveling stellar evolution, and estimating stellar ages.
Spectroscopic Parallax: Gauging Stellar Distances
One of the most ingenious applications of the HR Diagram lies in its ability to determine stellar distances through a technique known as spectroscopic parallax. Unlike geometric parallax, which is limited to relatively nearby stars, spectroscopic parallax can be applied to stars much farther away.
The process begins with obtaining a high-resolution spectrum of the target star.
By carefully analyzing the star's spectral lines, astronomers can determine its spectral type (e.g., G2) and luminosity class (e.g., V for main-sequence stars, III for giants).
This classification places the star on a specific location on the HR Diagram, allowing astronomers to infer its absolute magnitude.
Knowing the absolute magnitude (intrinsic brightness) and measuring the apparent magnitude (observed brightness from Earth), the distance to the star can be calculated using the distance modulus equation:
m - M = 5 log₁₀(d/10)
where m is the apparent magnitude, M is the absolute magnitude, and d is the distance in parsecs.
Spectroscopic parallax, while not as precise as geometric parallax for nearby stars, extends the reach of distance measurements far beyond what would otherwise be possible. It serves as a crucial rung on the cosmic distance ladder.
Stellar Evolution: Tracing Stellar Life Cycles
The HR Diagram is not merely a snapshot of the stellar population. It also provides invaluable insights into stellar evolution.
Stars do not remain static on the HR Diagram. As they age and exhaust their nuclear fuel, they migrate to different regions of the diagram, tracing evolutionary tracks that reveal their life stories.
Main sequence stars, fusing hydrogen into helium in their cores, spend the majority of their lives in a relatively stable location on the HR Diagram.
However, as hydrogen fuel dwindles, stars evolve off the main sequence, expanding into giants or supergiants.
These evolved stars occupy distinct regions on the HR Diagram, allowing astronomers to observe stellar evolution in real-time across vast stellar populations. The study of open and globular clusters, each representing coeval populations of stars, allows astronomers to observe many evolutionary stages at once.
The distribution of stars within a cluster on the HR Diagram provides crucial evidence for understanding the timeline and pathways of stellar evolution.
Isochrone Fitting: Determining Stellar Ages
Building upon its utility in tracing stellar evolution, the HR Diagram also provides a means of estimating the ages of stellar populations through a technique known as isochrone fitting.
An isochrone is a theoretical curve on the HR Diagram that represents the predicted locations of stars of the same age but different masses.
By comparing the observed distribution of stars in a cluster or stellar population with a series of theoretical isochrones, astronomers can determine the age of the population.
The "turn-off point," the point on the main sequence where stars begin to evolve off towards the giant branch, is particularly sensitive to age.
Older clusters exhibit a turn-off point at lower luminosities and temperatures, indicating that more massive stars have already exhausted their core hydrogen fuel.
Isochrone fitting is a complex process involving sophisticated stellar models and careful consideration of factors such as metallicity and distance.
However, when applied rigorously, it provides a powerful tool for dating stellar populations and reconstructing the history of galaxies and the universe.
In conclusion, the HR Diagram transcends its initial purpose as a classification tool, serving as a cornerstone of modern astrophysics. Through techniques like spectroscopic parallax, the tracing of stellar evolution, and isochrone fitting, it unlocks profound insights into the vastness of space, the life cycles of stars, and the age of the cosmos.
Physical Parameters: Connecting Spectra to Reality
Following the foundational groundwork laid by early astronomical pioneers, the establishment of a standardized system for classifying stars became paramount. The Harvard Spectral Classification system emerged as the definitive framework. It offered a structured method for categorizing stars. However, spectral type alone is but one piece of a much larger puzzle. Fully understanding a star requires connecting observable spectral characteristics to tangible physical properties, such as temperature.
The Intimate Relationship Between Spectral Type and Effective Temperature
At the heart of stellar classification lies a profound relationship: the connection between a star's spectral type and its effective temperature. Spectral type, designated by the iconic OBAFGKM sequence, isn't merely an arbitrary categorization. Rather, it is an indicator of a star's surface temperature.
O-type stars, blazing with extreme heat, represent the high-temperature end of the spectrum. M-type stars, with their comparatively cooler surfaces, occupy the other end.
Each spectral class represents a specific temperature range. The observed spectral features, notably the presence and strength of specific absorption lines, directly correlate with this temperature. This correlation allows astronomers to estimate a star's surface temperature from its spectral classification.
Blackbody Radiation: Unveiling the Energetic Signature
To understand this relationship more deeply, we must consider the principles of blackbody radiation. A star, to a first approximation, can be modeled as a blackbody, an object that absorbs all electromagnetic radiation incident upon it. A blackbody emits radiation across all wavelengths, with the intensity and distribution of this radiation dictated solely by its temperature.
The Stefan-Boltzmann Law dictates that the total energy radiated per unit surface area of a blackbody is proportional to the fourth power of its absolute temperature (E = σT⁴). This means that even small changes in temperature result in significant changes in emitted energy.
Wien's Displacement Law further refines this concept. It stipulates that the wavelength at which a blackbody emits the most radiation is inversely proportional to its temperature. Hotter stars emit predominantly at shorter, bluer wavelengths, while cooler stars emit primarily at longer, redder wavelengths. The spectral energy distribution, essentially a graph of energy output versus wavelength, provides a detailed profile of a star's blackbody radiation. Analyzing this distribution allows astronomers to derive an accurate measurement of the star's effective temperature.
Temperature's Influence on Stellar Spectra: Ionization and Excitation
Temperature exerts a powerful influence on the composition and appearance of stellar spectra. Specifically, it governs the ionization and excitation states of elements within a star's atmosphere.
High temperatures cause atoms to become ionized, stripping away electrons and altering their ability to absorb specific wavelengths of light. For example, O-type stars, with their scorching temperatures, exhibit spectra dominated by lines from highly ionized elements like helium and silicon.
Conversely, cooler stars have more neutral atoms, resulting in spectra rich in lines from neutral metals and even molecules. The strength of these absorption lines acts as a thermometer. Careful analysis of the line intensities enables astronomers to determine the temperature and chemical composition of a stellar atmosphere. Understanding these complex interactions is crucial for accurately interpreting stellar spectra and extracting meaningful physical parameters.
Stellar Composition and Metallicity: The Building Blocks of Stars
Following the foundational groundwork laid by early astronomical pioneers, the establishment of a standardized system for classifying stars became paramount. The Harvard Spectral Classification system emerged as the definitive framework. It offered a structured method for categorizing stars. However, understanding the chemical composition of these celestial objects was equally critical. This endeavor unveiled not only what stars are made of, but also how their composition influences their life cycle and ultimate fate.
Unveiling the Elemental Makeup: Payne-Gaposchkin's Breakthrough
In the early 20th century, a pivotal discovery reshaped our understanding of stellar interiors. Cecilia Payne-Gaposchkin, through her groundbreaking doctoral thesis, demonstrated that hydrogen and helium are, in fact, the dominant elements in stars.
This revelation was initially met with skepticism, as it contradicted prevailing scientific beliefs. However, her meticulous analysis of stellar spectra provided compelling evidence. It established that these lightweight elements constitute the vast majority of stellar mass.
Payne-Gaposchkin's work not only corrected previous misconceptions, but also laid the foundation for modern stellar astrophysics. It illuminated the fundamental chemical uniformity of stars across the galaxy.
Metallicity: More Than Just Metals
While hydrogen and helium comprise the bulk of stellar material, the presence of heavier elements, collectively termed "metals" in astronomical parlance, plays a crucial role. Metallicity refers to the abundance of elements heavier than hydrogen and helium within a star. It's a key parameter that influences stellar evolution and characteristics.
Stars are born from interstellar clouds. The clouds contain remnants of previous generations of stars. The metallicity of a star reflects the chemical composition of the gas cloud from which it formed.
The Nuances of Metallicity Measurement
Metallicity is typically expressed as a ratio relative to the Sun's metallicity. This allows astronomers to compare the chemical composition of different stars. Higher metallicity implies a greater proportion of heavier elements. It contrasts with lower metallicity, indicating a relatively pristine composition closer to the primordial universe.
The Impact of Metallicity on Stellar Evolution
Metallicity significantly affects various aspects of stellar evolution. It shapes stellar structure, lifespan, and eventual fate.
Higher metallicity can lead to increased opacity in stellar interiors. This in turn alters the rate of energy transport and nuclear fusion.
Shaping Stellar Structure
Metals contribute to the opacity of stellar gas. This makes it more difficult for radiation to escape from the core. The increased opacity leads to a higher core temperature and a steeper temperature gradient within the star.
Consequently, stars with higher metallicities tend to be more compact and have smaller radii. Their internal structure differs significantly from their metal-poor counterparts.
Altering Lifespan and Fate
Metallicity also influences the rate of nuclear fusion reactions within the stellar core. Higher metallicity can catalyze certain fusion processes, accelerating the consumption of nuclear fuel.
As a result, metal-rich stars tend to have shorter lifespans compared to metal-poor stars of the same mass. They may also follow different evolutionary pathways, potentially leading to the formation of different types of stellar remnants, such as white dwarfs, neutron stars, or black holes.
Population I, II, and III Stars: A Metallicity-Based Classification
Based on metallicity, stars can be broadly classified into different populations:
- Population I stars are metal-rich. These are typically found in the spiral arms of galaxies. They are younger and formed from gas clouds enriched by previous generations of stars.
- Population II stars are metal-poor. They reside in globular clusters and the galactic halo. These stars are older and formed from gas clouds that had not yet been significantly enriched with heavy elements.
- Population III stars are theorized to be the very first stars that formed in the universe. They are predicted to be extremely metal-poor (or even metal-free) and very massive. They have not yet been directly observed.
Understanding stellar composition and metallicity provides crucial insights into the formation, evolution, and ultimate fate of stars. This knowledge helps us to piece together the puzzle of our universe's history.
Tools of the Trade: Telescopes, Spectrographs, and Filters
Following the foundational groundwork laid by early astronomical pioneers, the establishment of a standardized system for classifying stars became paramount. The Harvard Spectral Classification system emerged as the definitive framework. It offered a structured method for categorizing stars based on their spectral characteristics. This analytical work relies heavily on specialized instruments.
These instruments allow scientists to gather and interpret the light emitted by these distant celestial bodies. Telescopes, spectrographs, and filters are essential tools for astronomers seeking to unravel the mysteries of stellar spectra. These tools make precise classifications possible.
The Indispensable Spectrograph
At the heart of stellar classification lies the spectrograph. It is an instrument designed to disperse light into its constituent wavelengths. It creates a spectrum that reveals the unique fingerprint of a star.
The spectrograph's importance is undeniable. Without it, we would be unable to discern the intricate details hidden within stellar light. The spectral lines tell us about a star's chemical composition, temperature, density, and velocity.
Capturing Light: The Telescope's Role
Telescopes, whether ground-based or space-borne, serve as the primary light-gathering instruments in astronomy. Their primary function is to collect as much light as possible from distant stars.
The aperture size of a telescope directly impacts its light-gathering ability. Larger apertures allow astronomers to observe fainter and more distant objects. Advanced telescopes, such as the James Webb Space Telescope, extend our observational capabilities. They provide unparalleled views of the cosmos.
Fine-Tuning Observations with Filters
Filters play a crucial role in isolating specific wavelengths of light. They enhance our understanding of stellar phenomena. By selectively transmitting certain colors of light, filters enable astronomers to:
- Study specific spectral features.
- Reduce unwanted background noise.
- Enhance the contrast of images.
Types of Filters
Various types of filters exist, each serving a unique purpose:
- Broadband filters are useful for capturing general color information.
- Narrowband filters isolate specific emission lines, such as those from hydrogen or oxygen.
- Specialized filters (e.g., polarizing filters) provide additional information about the properties of light.
The strategic use of filters allows for more precise and detailed spectral analysis. This is a key step in determining a star's classification and characteristics.
Video: Sun Spectral Type: A Beginner's Guide
FAQs: Sun Spectral Type: A Beginner's Guide
What does a star's spectral type tell us?
A star's spectral type is a classification based on its surface temperature and the elements present in its atmosphere. By examining the spectrum of light emitted, we can learn a lot. Since the sun is a star, its sun spectral type reveals key information about its characteristics.
What is the sun's spectral type and what does it mean?
The sun's spectral type is G2V. The "G2" signifies its surface temperature, roughly 5,778 Kelvin, and the "V" indicates that it's a main-sequence star, meaning it's in the stable, hydrogen-burning phase of its life. So the sun spectral type places it among other stars with similar properties.
How is a star's spectral type determined?
A star's spectral type is primarily determined by analyzing the absorption lines in its spectrum. These dark lines correspond to elements that absorb specific wavelengths of light. The pattern of these lines, along with the star's overall color, reveals the star's temperature and composition, directly impacting sun spectral type assessments too.
Why is understanding the sun spectral type important?
Knowing the sun spectral type helps us understand its energy output, life cycle stage, and its potential impact on Earth. It also allows us to compare our sun to other stars in the galaxy and better understand stellar evolution in general. A star's sun spectral type provides a wealth of information.
So, the next time you're soaking up some sun (don't forget the sunscreen!), remember that our star is a G2V, a perfectly average member of the main sequence club. Understanding the Sun's spectral type might seem like astrophysics jargon, but hopefully, you now have a better appreciation for where our sun fits into the grand cosmic picture!