Sensible Heat vs Latent Heat: Home Comfort
Understanding the principles of sensible heat vs latent heat is crucial for optimizing home comfort, especially when considering the performance of HVAC systems. The American Society of Heating, Refrigerating and Air-Conditioning Engineers (ASHRAE) defines sensible heat as heat that changes the temperature of a substance without changing its state, while latent heat involves a change in state, such as water evaporating in a humidifier. Indoor climate control heavily relies on managing both types of heat; for example, a central air conditioner must remove both the sensible heat, lowering the air temperature, and the latent heat, reducing humidity levels. Homeowners can use tools such as a psychrometric chart to visualize the relationship between temperature, humidity, and enthalpy, allowing them to better understand and manage their thermal environment.
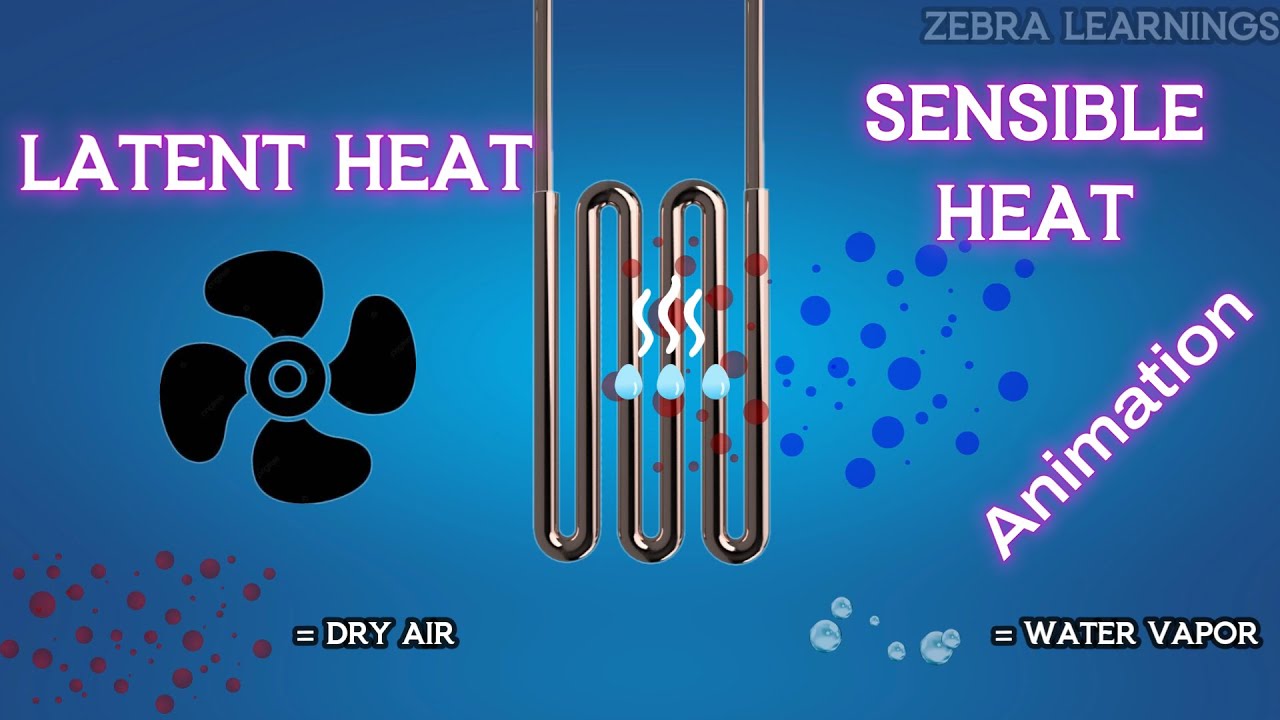
Image taken from the YouTube channel Zebra Learnings , from the video titled Latent Heat and Sensible Heat Explained | Humidity | Animation | #hvac #hvacsystem #hvacmaintenance .
Heat transfer and thermodynamics are foundational pillars in understanding energy behavior. Grasping their principles is critical for various fields, from engineering to environmental science. This section lays the groundwork, defining key concepts and fundamental laws governing energy and heat.
Defining Heat: Sensible vs. Latent
Heat, at its core, is energy in transit, flowing due to temperature differences. However, heat's effects manifest differently depending on how it interacts with matter. This leads to the distinction between sensible and latent heat.
Sensible Heat: The Temperature Raiser
Sensible heat refers to heat transfer that causes a change in temperature of a substance. This temperature change is directly measurable using a thermometer.
For instance, the increase in temperature of water when it is heated on a stove is an example of sensible heat. Similarly, the warming of a metal bar when placed in a hot environment demonstrates sensible heat transfer. The key here is that the substance's physical state remains unchanged.
Latent Heat: The Phase Changer
Latent heat, on the other hand, is heat transfer that results in a phase change of a substance, without any change in temperature. This involves transitioning between solid, liquid, and gaseous states.
Consider ice melting into water at 0°C. The addition of heat doesn't raise the temperature; instead, it breaks the bonds holding the ice molecules together. This heat is latent heat of fusion.
Similarly, when water boils at 100°C, the added heat transforms the liquid into steam, without increasing the temperature of the water. This is the latent heat of vaporization.
These phase transitions are crucial in many natural and industrial processes, influencing everything from weather patterns to material processing.
Thermodynamics: The Science of Energy Transformation
Thermodynamics is the broad science that governs heat transfer and energy transformations. It dictates the rules for how energy interacts with matter and the constraints on these interactions.
The First Law: Conservation of Energy
The First Law of Thermodynamics is the principle of conservation of energy. This law states that energy cannot be created or destroyed; it can only be transformed from one form to another.
In simpler terms, the total energy in an isolated system remains constant. When heat is added to a system, it either increases the system's internal energy or performs external work, or both. This law is fundamental to understanding energy balances in any process.
The Second Law of Thermodynamics introduces the concept of entropy and defines the direction of heat flow. Entropy is often described as the measure of disorder or randomness in a system.
The Second Law states that heat naturally flows from a hotter object to a colder object. This flow is accompanied by an increase in entropy in the system.
Reversing this process, making heat flow spontaneously from cold to hot, would require external work. The Second Law highlights the inherent directionality of many natural processes. It explains why engines can't be perfectly efficient and why energy conversions always involve some loss as waste heat.
Principles of Heat Transfer: Understanding the Process
Heat transfer and thermodynamics are foundational pillars in understanding energy behavior. Grasping their principles is critical for various fields, from engineering to environmental science. This section lays the groundwork, defining key concepts and fundamental laws governing energy and heat.
What is Heat Transfer?
At its core, heat transfer is the movement of thermal energy from one location to another, driven by a temperature difference, also known as a temperature gradient.
This movement occurs naturally, with heat flowing from regions of higher temperature to regions of lower temperature.
This process continues until thermal equilibrium is reached, meaning the temperatures are equalized, and there is no longer a net flow of thermal energy.
Understanding this fundamental concept is crucial for analyzing various phenomena, from the cooling of electronic devices to the heating of buildings.
Modes of Heat Transfer
There are three primary modes of heat transfer: conduction, convection, and radiation. Each operates via distinct mechanisms and is influenced by different material properties and environmental conditions.
Conduction
Conduction is the transfer of heat through a material via direct contact and molecular vibration. It is most efficient in solids where molecules are tightly packed.
Imagine holding a metal spoon in a hot bowl of soup. The heat from the soup is transferred to the spoon through molecular vibrations.
The hotter molecules in the soup collide with the cooler molecules of the spoon, transferring their kinetic energy.
Thermal conductivity is a key property defining a material's ability to conduct heat. Materials with high thermal conductivity, like metals, readily transfer heat, while materials with low thermal conductivity, like insulators, resist heat transfer.
Convection
Convection involves heat transfer through the movement of fluids, either liquids or gases.
As a fluid heats up, it becomes less dense and rises, while cooler, denser fluid sinks, creating a cycle of movement that transfers heat.
There are two main types of convection: natural and forced.
Natural convection occurs due to density differences caused by temperature variations, like boiling water.
Forced convection utilizes external means, such as a fan or pump, to circulate the fluid and enhance heat transfer, such as in a car radiator.
Radiation
Radiation is the transfer of heat through electromagnetic waves. Unlike conduction and convection, radiation does not require a medium to propagate, enabling heat transfer through a vacuum.
The sun warming the earth is a prime example of radiative heat transfer. The energy from the sun travels through space as electromagnetic waves, warming the earth's surface upon absorption.
All objects emit thermal radiation, with the amount and wavelength of radiation depending on the object's temperature and surface properties.
Emissivity is a key property that dictates how effectively a surface emits thermal radiation. A black body, for example, has an emissivity of 1 and is a perfect emitter and absorber of radiation.
Measurement and Properties: Quantifying Thermal Energy
Understanding the principles of heat transfer requires not only a grasp of the fundamental concepts but also the ability to quantify thermal energy and the properties of materials that govern its flow. This section delves into the essential measurements and material characteristics that determine how heat interacts with its environment. We will explore temperature scales, energy units, and the critical material properties of specific heat capacity and latent heat.
Temperature: Measuring Thermal Energy
Temperature is the fundamental measure of thermal energy. It provides a way to quantify the average kinetic energy of the molecules within a substance. Higher temperatures indicate that molecules are moving faster and possess greater kinetic energy.
Temperature Scales: Celsius, Fahrenheit, and Kelvin
Different temperature scales have been developed for various applications. The most common are Celsius (°C), Fahrenheit (°F), and Kelvin (K).
-
Celsius (°C): Based on the freezing point (0°C) and boiling point (100°C) of water.
-
Fahrenheit (°F): Based on the freezing point (32°F) and boiling point (212°F) of water. This scale is primarily used in the United States.
-
Kelvin (K): The absolute temperature scale, where 0 K represents absolute zero, the theoretical point at which all molecular motion ceases. The Kelvin scale is widely used in scientific applications.
Conversion Formulas:
- °F = (°C × 9/5) + 32
- °C = (°F - 32) × 5/9
- K = °C + 273.15
Temperature and Kinetic Energy
Temperature is directly proportional to the average kinetic energy of the molecules in a substance. In other words, as temperature increases, the average speed of the molecules also increases. This relationship is fundamental to understanding how heat transfer occurs at a molecular level.
Energy Units and Measurement
Energy, including thermal energy, is measured in various units, each with its specific application. Understanding these units is crucial for quantifying heat transfer processes.
British Thermal Unit (BTU)
The British Thermal Unit (BTU) is defined as the amount of energy required to raise the temperature of one pound of water by one degree Fahrenheit. It is commonly used in the United States to measure the heating or cooling capacity of HVAC systems.
Joule (J)
The Joule (J) is the standard unit of energy in the International System of Units (SI). It is defined as the amount of work done when a force of one newton displaces a mass by one meter in the direction of the force.
Calorie (cal)
The Calorie (cal) is defined as the amount of energy required to raise the temperature of one gram of water by one degree Celsius. A kilocalorie (kcal), often referred to simply as "Calorie" (with a capital C), is equal to 1000 calories and is commonly used to measure the energy content of food.
Material Properties Affecting Heat Transfer
The properties of a material significantly influence how it conducts, stores, and releases thermal energy.
Specific Heat Capacity (c)
Specific heat capacity (c) is the amount of heat required to raise the temperature of one unit mass of a substance by one degree. It is a measure of a substance's ability to store thermal energy. Materials with a high specific heat capacity require more energy to change their temperature compared to materials with a low specific heat capacity.
-
High Specific Heat Capacity: Water has a high specific heat capacity, making it effective at storing thermal energy.
-
Low Specific Heat Capacity: Metals like copper and aluminum have low specific heat capacities, meaning they heat up and cool down quickly.
Latent Heat of Fusion
The latent heat of fusion is the energy required to change a substance from a solid to a liquid state at its melting point, without changing its temperature. This energy is used to break the intermolecular bonds that hold the solid structure together.
- Example: Ice melting into water requires energy input, even though the temperature remains at 0°C during the melting process.
Latent Heat of Vaporization
The latent heat of vaporization is the energy required to change a substance from a liquid to a gas state at its boiling point, without changing its temperature. This energy is used to overcome the intermolecular forces that hold the liquid together.
- Example: Boiling water requires a significant amount of energy, even though the temperature remains at 100°C during the boiling process. The steam produced stores the latent heat, which is released upon condensation.
Phase Changes: Transforming Matter with Heat
Understanding the principles of heat transfer extends beyond the simple movement of thermal energy; it also encompasses the transformations of matter itself. Phase changes, the transitions between solid, liquid, and gaseous states, are fundamental processes driven by the addition or removal of heat. Each phase change is associated with a specific amount of energy, and these transitions play crucial roles in various natural and technological applications.
Detailed Explanation of Phase Changes
Phase changes are physical processes where a substance transitions from one state of matter to another. These transitions occur when the energy of the substance changes, usually through the addition or removal of heat.
Solid-Liquid: Melting and Freezing
Melting is the process by which a solid transforms into a liquid. This occurs when the solid absorbs heat, increasing the kinetic energy of its molecules until they overcome the intermolecular forces holding them in a fixed arrangement. The temperature at which melting occurs is known as the melting point.
Freezing is the reverse process, where a liquid transforms into a solid. This happens when the liquid releases heat, reducing the kinetic energy of its molecules and allowing them to form a rigid structure. The temperature at which freezing occurs is known as the freezing point, which, for pure substances, is the same as the melting point.
Both melting and freezing involve the latent heat of fusion, which is the energy required to change the phase without changing the temperature. Examples include ice melting into water at 0°C and liquid water freezing into ice at 0°C.
Liquid-Gas: Boiling and Condensation
Boiling is the process by which a liquid transforms into a gas. This occurs when the liquid absorbs heat, increasing the kinetic energy of its molecules until they overcome the intermolecular forces holding them together in the liquid state. The temperature at which boiling occurs is known as the boiling point.
Condensation is the reverse process, where a gas transforms into a liquid. This happens when the gas releases heat, reducing the kinetic energy of its molecules and allowing them to form a liquid.
Boiling and condensation involve the latent heat of vaporization, which is the energy required to change the phase without changing the temperature. Factors affecting the boiling point include pressure; higher pressures generally lead to higher boiling points.
Examples include water boiling into steam at 100°C (at standard atmospheric pressure) and steam condensing into water.
Solid-Gas: Sublimation and Deposition
Sublimation is the process by which a solid transforms directly into a gas, bypassing the liquid phase. This occurs when the solid absorbs enough heat to overcome the intermolecular forces holding its molecules together.
Deposition is the reverse process, where a gas transforms directly into a solid, bypassing the liquid phase. This happens when the gas releases heat, allowing its molecules to form a solid structure directly.
A common example of sublimation is dry ice (solid carbon dioxide) sublimating into gaseous carbon dioxide. Deposition is exemplified by frost forming on a cold surface, where water vapor in the air freezes directly into ice crystals.
Humidity and Air Properties: Understanding Atmospheric Moisture
Phase Changes: Transforming Matter with Heat Understanding the principles of heat transfer extends beyond the simple movement of thermal energy; it also encompasses the transformations of matter itself. Phase changes, the transitions between solid, liquid, and gaseous states, are fundamental processes driven by the addition or removal of heat. Each...
Beyond the principles governing conduction, convection, and radiation, the properties of air, especially its moisture content, play a pivotal role in thermal comfort and environmental processes. Humidity, dew point, and the latent heat associated with water's phase transitions profoundly influence our perception of temperature and the dynamics of weather systems.
Relative Humidity: A Measure of Air's Moisture Capacity
Relative humidity (RH) is a crucial metric for gauging the amount of moisture present in the air, expressed as a percentage of the maximum amount of moisture the air can hold at a given temperature. It's not simply a measure of how much water is in the air, but rather a measure of how saturated the air is with water vapor.
Higher relative humidity hinders evaporation, making warm days feel even hotter because our bodies rely on evaporation to cool down. This explains why a day at 85°F (29°C) with 80% RH feels significantly more oppressive than a day at the same temperature with 30% RH.
Calculating and Influencing Relative Humidity
Relative humidity is calculated as the ratio of the partial pressure of water vapor in the air to the saturation vapor pressure at that temperature, multiplied by 100%.
Several factors influence relative humidity:
- Temperature: Warmer air can hold more moisture than colder air. As temperature increases, relative humidity decreases (assuming the amount of water vapor remains constant).
- Water Vapor Content: An increase in the amount of water vapor in the air will directly increase the relative humidity.
- Evaporation: Evaporation increases the moisture content of the air, directly increasing relative humidity.
- Condensation: Condensation (the reverse of evaporation) decreases the water vapor in the air, lowering relative humidity.
Dew Point: The Condensation Threshold
The dew point is the temperature to which air must be cooled, at constant pressure, for water vapor to condense into liquid water. It is a direct measure of the absolute amount of moisture in the air.
Unlike relative humidity, which is temperature-dependent, the dew point provides a more stable indicator of humidity levels.
Dew Point, Relative Humidity, and Air Temperature: A Connected Trio
The dew point, relative humidity, and air temperature are intricately linked. When the air temperature equals the dew point, the relative humidity is 100%, and condensation begins to occur.
This is why dew forms on grass during cool mornings – the ground cools overnight, lowering the air temperature to the dew point, causing water vapor to condense on surfaces.
The closer the dew point is to the air temperature, the higher the relative humidity and the greater the likelihood of precipitation, fog, or the formation of dew.
The Role of Water (H₂O) in Latent Heat Processes
Water (H₂O) plays a pivotal role in latent heat processes, especially within the atmosphere. Evaporation and condensation, both phase transitions of water, involve significant energy transfer without a change in temperature.
This energy, known as latent heat, is either absorbed during evaporation or released during condensation.
Evaporation, Condensation, and Weather Patterns
Evaporation absorbs energy from the surroundings, cooling the environment. Conversely, condensation releases energy, warming the environment. This cycle is crucial in regulating global temperatures and driving weather patterns.
- Evaporation from oceans and lakes: This process absorbs vast amounts of energy from the sun, providing the energy that fuels storms and moderates coastal climates.
- Condensation in clouds: As water vapor condenses in clouds, it releases latent heat, providing energy for cloud development and precipitation. The heat released during condensation can also contribute to the intensification of storms.
Understanding these properties of air, including humidity, dew point, and the latent heat associated with water's phase changes, is essential for creating comfortable living environments and comprehending the complex dynamics of our planet's climate system.
Applications in HVAC Systems: Controlling Heat Transfer for Comfort
Humidity and Air Properties: Understanding Atmospheric Moisture Phase Changes: Transforming Matter with Heat Understanding the principles of heat transfer extends beyond the simple movement of thermal energy; it also encompasses the transformations of matter itself. Phase changes, the transitions between solid, liquid, and gaseous states, are funda...
Heat transfer principles are not just theoretical concepts; they are the very foundation upon which our modern climate control systems operate. HVAC (Heating, Ventilation, and Air Conditioning) systems are prime examples of how we harness these principles to create comfortable and healthy indoor environments.
HVAC: A Symphony of Thermal Management
HVAC systems represent a comprehensive approach to building climate control. They orchestrate temperature, humidity, and air quality, ensuring optimal conditions for occupants.
HVAC isn't merely about comfort; it's a cornerstone of health and productivity. Controlled environments reduce the spread of airborne illnesses, improve cognitive function, and enhance overall well-being. Furthermore, efficient HVAC systems significantly reduce energy consumption, leading to cost savings and a smaller environmental footprint.
Core Components and Systems
Let's delve into the core components that drive HVAC systems, focusing on air conditioners and heat pumps, which are arguably the most important components.
Air Conditioners: Mastering the Refrigeration Cycle
Air conditioners are designed to remove heat and moisture from indoor air, creating a cooler and drier environment. They achieve this through a closed-loop system utilizing a refrigerant. The refrigerant absorbs heat as it evaporates, and releases heat as it condenses.
The Refrigeration Cycle: A Step-by-Step Breakdown
The refrigeration cycle is a four-stage process:
- Evaporation: Refrigerant evaporates in the evaporator coil, absorbing heat from the indoor air and cooling it.
- Compression: The refrigerant vapor is compressed, increasing its temperature and pressure.
- Condensation: The hot, high-pressure refrigerant condenses in the condenser coil, releasing heat to the outside air.
- Expansion: The high-pressure liquid refrigerant expands through an expansion valve, reducing its pressure and temperature, preparing it to repeat the cycle.
Heat Pumps: Dual-Purpose Thermal Regulators
Heat pumps offer a versatile solution for both heating and cooling. Unlike traditional furnaces that generate heat, heat pumps transfer heat from one location to another.
In cooling mode, a heat pump operates like an air conditioner, extracting heat from the indoor air and releasing it outdoors. In heating mode, the cycle is reversed; the heat pump extracts heat from the outside air (even in cold temperatures) and transfers it indoors.
Reversing the Cycle: A Clever Engineering Feat
The key to a heat pump's dual functionality lies in a reversing valve. This valve allows the refrigerant flow to be reversed, changing the direction of heat transfer. This clever design allows heat pumps to provide efficient heating and cooling with a single piece of equipment, making them a popular choice for energy-conscious consumers.
Comfort and Energy Efficiency: Optimizing for Well-being and Sustainability
Understanding the principles of heat transfer extends beyond the simple movement of thermal energy; it also encompasses the transformations of matter itself. Phase changes, humidity, and air properties are key factors in achieving comfortable and energy-efficient environments. This section will delve into the concept of the comfort zone, its determining factors, and strategies for maximizing energy efficiency in buildings.
Defining the Human Comfort Zone
The "comfort zone" is a complex interplay of environmental conditions where the majority of occupants experience thermal satisfaction.
It is not a fixed point but rather a range influenced by various factors that interact to affect our perception of warmth and coolness.
Understanding and managing these factors is essential for designing HVAC systems and buildings that promote both well-being and energy conservation.
Factors Influencing Thermal Comfort
Environmental Factors
The core environmental variables that define the comfort zone are:
-
Air Temperature: The most obvious factor, influencing heat gain or loss through convection and radiation.
-
Radiant Temperature: The temperature of surrounding surfaces, significantly impacting radiant heat exchange.
-
Humidity: Affects the rate of evaporative cooling from the skin, thus playing a critical role in perceived temperature.
-
Air Velocity: Influences convective heat transfer and evaporation, with higher velocities generally increasing cooling.
Personal Factors
Beyond environmental parameters, individual characteristics also play a key role:
-
Clothing: Acts as insulation, affecting heat loss. Thicker clothing shifts the comfort zone to cooler temperatures.
-
Activity Level: Metabolic rate increases with activity, generating more internal heat and altering thermal needs.
-
Individual Preferences: Subjective factors like acclimatization, health status, and personal biases can significantly vary comfort perception. This introduces complexity in defining universally "comfortable" environments.
Energy Efficiency in Buildings: Minimizing Unwanted Heat Transfer
Buildings act as thermal systems, constantly exchanging heat with their surroundings. Minimizing unwanted heat transfer is paramount for energy efficiency and reducing the load on HVAC systems.
Insulation
Effective insulation is the cornerstone of energy-efficient building design. It reduces conductive heat transfer through walls, roofs, and floors.
Different materials offer varying levels of insulation (R-value), and selecting the right insulation is vital for a building's overall thermal performance.
Air Sealing
Air leaks, often overlooked, can be a significant source of energy loss. Sealing cracks and gaps in the building envelope minimizes air infiltration, reducing both heat loss in winter and heat gain in summer.
Effective air sealing is often more cost-effective than simply adding more insulation.
Windows
Windows are typically the weakest link in a building's thermal envelope.
-
Energy-efficient windows with multiple panes, low-emissivity (low-E) coatings, and gas fills can significantly reduce heat transfer.
-
Proper window orientation and shading strategies can also minimize solar heat gain during warmer months.
Shading
Shading devices, such as awnings, overhangs, and trees, block direct sunlight and reduce solar heat gain.
Effective shading can substantially reduce cooling loads, particularly during peak summer months.
Optimizing HVAC Systems for Energy Efficiency
Even with a well-insulated and sealed building envelope, HVAC systems are necessary for maintaining comfortable indoor conditions. Optimizing their performance is crucial for minimizing energy consumption.
Programmable Thermostats
Programmable thermostats allow for automated temperature adjustments based on occupancy schedules, reducing energy waste during unoccupied periods.
This seemingly simple measure can result in significant energy savings over time.
Regular Maintenance
Regular maintenance, including filter changes, coil cleaning, and system inspections, ensures that HVAC systems operate at peak efficiency.
Neglecting maintenance can lead to reduced performance and increased energy consumption.
Energy-Efficient Equipment
Choosing energy-efficient HVAC equipment, such as models with high Seasonal Energy Efficiency Ratio (SEER) and Heating Seasonal Performance Factor (HSPF), can dramatically lower energy bills.
While initial costs might be higher, the long-term energy savings often outweigh the upfront investment.
Video: Sensible Heat vs Latent Heat: Home Comfort
FAQs: Sensible Heat vs Latent Heat in Home Comfort
How does sensible heat affect my home's temperature?
Sensible heat is the energy that causes a change in temperature you can feel. In your home, it directly impacts how hot or cold the air is. Increasing sensible heat raises the air temperature, while decreasing it lowers the temperature. Managing sensible heat is key to keeping your home at a comfortable temperature.
What role does latent heat play in home humidity?
Latent heat is the energy absorbed or released during a change in state, like water evaporating into vapor (humidity). In your home, latent heat is primarily related to humidity. Air conditioning systems remove latent heat by condensing water vapor, reducing humidity and making your home feel more comfortable even at the same temperature.
Why can my thermostat say one temperature, but I still feel uncomfortable?
Your thermostat measures sensible heat, indicating the air temperature. However, high humidity (related to latent heat) can make you feel hotter than the thermostat reads. This is because high humidity hinders sweat evaporation, which is your body's natural cooling mechanism. Managing both sensible heat and latent heat is important for overall comfort.
How can I improve my home's comfort by addressing both sensible heat and latent heat?
You can improve comfort by ensuring your HVAC system is properly sized and functioning. Proper sizing handles sensible heat effectively. Features like dehumidifiers or air conditioners with good dehumidification capabilities can remove excess moisture, addressing latent heat. This combination provides optimal comfort at a chosen temperature.
So, the next time you're fiddling with your thermostat or scratching your head about why your house feels humid even though the temperature is okay, remember the difference between sensible heat vs latent heat. Understanding these two types of heat can really help you dial in your home comfort and maybe even save a bit on your energy bill. Happy adjusting!