Unlock S Phase: The Secret To Perfect Cell Division (Explained)
Cellular replication, a fundamental process in biology, relies heavily on the accurate duplication of genetic material. DNA polymerase, a key enzyme, plays a critical role in this process, ensuring faithful replication during the s phase. The nucleolus, the site of ribosome biogenesis, contributes to the cell's capacity for DNA synthesis, which is so important to s phase. Dysfunction in the cell cycle checkpoints, mechanisms that monitor and regulate cell division progression, can lead to errors during DNA replication, impacting the integrity of the s phase. Understanding the intricacies of s phase, especially in relation to entities like DNA polymerase and cell cycle checkpoints, is paramount for comprehending cellular growth and division.
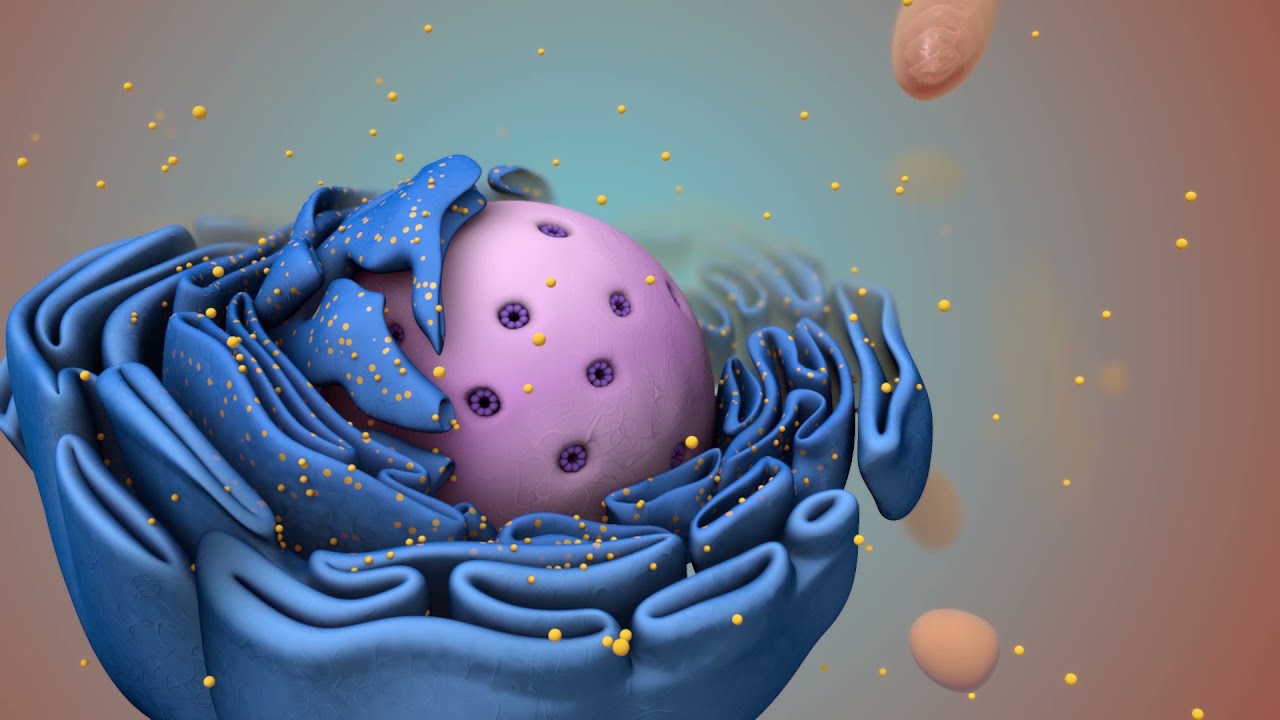
Image taken from the YouTube channel Nucleus Biology , from the video titled The Cell Cycle .
The life of a cell is a carefully orchestrated sequence of events known as the cell cycle. This cycle encompasses all stages of a cell’s existence, from its birth through growth, DNA replication, and ultimately, division into two daughter cells. Understanding the cell cycle is fundamental to comprehending life itself.
The Cell Cycle: A Symphony of Growth and Division
The cell cycle isn't just a random process; it's a highly regulated series of phases:
- G1 (Gap 1): A period of growth and preparation for DNA replication.
- S (Synthesis): Where DNA replication occurs.
- G2 (Gap 2): Further growth and preparation for cell division.
- M (Mitosis): Cell division itself.
Each phase plays a distinct and crucial role in ensuring the accurate and successful propagation of cellular life. Disruptions to any of these phases can have significant consequences for the cell.
S Phase: The Linchpin of Genetic Inheritance
Within this cycle, S phase, or Synthesis phase, stands out as the critical period where the cell meticulously duplicates its entire genome. This DNA replication process ensures that each daughter cell receives a complete and accurate copy of the genetic blueprint. Without accurate DNA replication, proper cell division wouldn't be possible.
S phase isn't just about making copies; it's about making perfect copies. The stakes are incredibly high.
The High Stakes of Accurate DNA Replication
Imagine the genome as an instruction manual. If the cell copies it with errors, the new cell won't have the proper instructions.
Accurate DNA replication is paramount for maintaining genetic integrity. Errors during this process can lead to mutations, which are permanent alterations in the DNA sequence. While some mutations may be harmless, others can disrupt normal cellular function.
The consequences of mutations can range from minor cellular malfunctions to severe diseases, including cancer. Cancer often arises from an accumulation of mutations that disrupt the delicate balance of cell growth and division.
Therefore, the fidelity of DNA replication during S phase is not just a cellular concern; it is a fundamental requirement for the health and well-being of the entire organism.
Unlocking S Phase: A Deep Dive into DNA Replication
The stakes are indeed high when considering the accuracy of DNA replication. An error-free genome duplication is not just desirable; it's absolutely essential for cellular survival and the prevention of disease. Now, let's unpack the complex molecular machinery and choreography that enables this remarkable feat of biological engineering during S phase.
The Orchestration of DNA Replication
DNA replication during S phase is a highly coordinated process involving a multitude of enzymes and proteins working in concert. This intricate dance ensures that the entire genome is faithfully duplicated before cell division.
Key Players in the Replication Process
Several key enzymes are essential for DNA replication.
DNA Polymerase: The Master Synthesizer
DNA polymerase is the central enzyme responsible for synthesizing new DNA strands. It adds nucleotides to the 3' end of a primer, using the existing DNA strand as a template.
DNA polymerase exhibits remarkable fidelity, ensuring that the newly synthesized strand is an accurate copy of the template.
Primase: The Initiator
Primase is an RNA polymerase that synthesizes short RNA primers. These primers provide a starting point for DNA polymerase to begin synthesizing new DNA strands.
Without primase, DNA replication cannot initiate.
Helicase: The Unzipping Enzyme
Helicase unwinds the DNA double helix at the replication fork, separating the two strands to provide access for DNA polymerase.
This unwinding process creates torsional stress ahead of the replication fork.
Topoisomerase: The Stress Reliever
Topoisomerase relieves the torsional stress created by helicase. It does so by cutting and rejoining DNA strands, preventing the DNA from becoming tangled or supercoiled.
Ligase: The Stitcher
Ligase joins Okazaki fragments on the lagging strand. These fragments are short DNA sequences synthesized discontinuously.
Ligase creates a phosphodiester bond between the fragments, forming a continuous DNA strand.
Leading vs. Lagging: A Tale of Two Strands
DNA replication is not a simple, continuous process on both strands of the DNA molecule.
The leading strand is synthesized continuously in the 5' to 3' direction, following the replication fork.
The lagging strand, however, is synthesized discontinuously in short fragments called Okazaki fragments, also in the 5' to 3' direction, but away from the replication fork. This is because DNA polymerase can only add nucleotides to the 3' end of a growing strand.
Stabilizing the Single Strands: The Role of SSBPs
Single-Stranded Binding Proteins (SSBPs) play a crucial role in stabilizing single-stranded DNA. By binding to the single strands, SSBPs prevent them from re-annealing or forming secondary structures, which could impede DNA replication.
Origin of Replication
DNA replication doesn't start at just one point.
Origins of replication are specific sites on the DNA molecule where replication begins. These sites are recognized by initiator proteins, which recruit the necessary enzymes to begin unwinding the DNA and synthesizing new strands. Eukaryotic chromosomes have multiple origins of replication to ensure rapid and efficient duplication of the large genome.
The Chromatin Landscape During S Phase: Remodeling for Replication
The meticulous work of DNA replication, orchestrated by enzymes like DNA polymerase and helicase, occurs within the context of chromatin. This intricate structure, composed of DNA and proteins, presents a significant challenge to the replication machinery. Understanding how chromatin is organized and dynamically remodeled during S phase is crucial to appreciating the fidelity and efficiency of DNA duplication.
Understanding Chromatin Structure
Chromatin is the complex assemblage of DNA and proteins that constitutes chromosomes within the nucleus of eukaryotic cells. Its primary function is to package long DNA molecules into a more compact, manageable form, preventing DNA entanglement and damage.
The fundamental repeating unit of chromatin is the nucleosome. This structure consists of approximately 147 base pairs of DNA wrapped around a core of eight histone proteins (two each of H2A, H2B, H3, and H4).
These nucleosomes are further organized into higher-order structures, ultimately forming the condensed chromosomes visible during cell division. The level of chromatin compaction directly influences DNA accessibility.
Histone Duplication and Assembly
As DNA is replicated during S phase, the cell must also duplicate its histone proteins to maintain chromatin structure on the newly synthesized DNA strands. This process involves a coordinated increase in histone production and their subsequent assembly.
Histone synthesis is tightly coupled with DNA replication. The cell employs several mechanisms to ensure that sufficient histone proteins are available when and where they are needed.
New histones are rapidly incorporated into nucleosomes behind the replication fork, working in conjunction with histone chaperone proteins. These chaperones guide the assembly process, preventing inappropriate histone aggregation and ensuring proper nucleosome spacing.
The pre-existing histones are distributed between the two daughter DNA molecules, and new histones fill in the gaps. This maintains epigenetic information, which is crucial for cell identity and function.
Chromatin Remodeling for Replication
The highly compacted nature of chromatin presents a barrier to the replication machinery. To overcome this obstacle, the cell employs chromatin remodeling complexes that transiently alter chromatin structure, allowing access to the DNA template.
These remodeling complexes utilize ATP hydrolysis to reposition, slide, or eject nucleosomes, creating regions of more accessible DNA. This dynamic remodeling is essential for DNA polymerase and other replication factors to gain access to the DNA.
Furthermore, histone modifications, such as acetylation and methylation, play a critical role in regulating chromatin accessibility. Acetylation generally promotes a more open chromatin state, facilitating replication, while methylation can have varied effects depending on the specific residue modified.
The precise interplay between chromatin remodeling complexes and histone modifications ensures that DNA replication occurs efficiently and accurately throughout the genome. These mechanisms are vital for maintaining genetic stability and preventing errors that could lead to disease.
The dance of DNA replication within the dynamically remodeled chromatin landscape is a feat of biological engineering. However, even with the best machinery, errors can arise. To safeguard the integrity of the genome, cells employ a sophisticated surveillance system.
Cell Cycle Checkpoints: Guardians of S Phase Fidelity
The cell cycle isn't a free-for-all; it's a highly regulated process. At strategic points, known as checkpoints, the cell assesses its readiness to proceed to the next phase. These checkpoints are critical control mechanisms that ensure the fidelity of DNA replication and cell division.
Checkpoints: Ensuring Accurate DNA Replication
Cell cycle checkpoints act as surveillance systems, monitoring the state of the cell and the accuracy of ongoing processes. They are crucial for preventing the replication of damaged DNA.
If errors or abnormalities are detected, the checkpoints trigger a halt in the cell cycle. This pause allows time for repair mechanisms to correct the problem before replication continues.
The Intra-S Phase Checkpoint: A Focus on DNA Replication Fidelity
The S phase checkpoint, also known as the intra-S checkpoint, is specifically responsible for monitoring DNA integrity during replication. It acts as a critical surveillance system, constantly scanning for DNA damage, stalled replication forks, and other replication errors.
This checkpoint is particularly important because DNA replication is a complex and error-prone process. Damage to DNA can arise spontaneously. Or it can arise from exposure to external agents like radiation or chemicals.
Mechanisms of S Phase Checkpoint Activation
The S phase checkpoint relies on a complex network of proteins. These proteins detect and respond to DNA damage and replication stress. Key players in this process include:
-
ATM and ATR kinases: These kinases are activated by DNA damage and stalled replication forks, respectively.
-
CHK1 and CHK2 kinases: ATM and ATR activate downstream kinases, CHK1 and CHK2, which then phosphorylate target proteins to halt the cell cycle.
These kinases initiate signaling cascades that ultimately lead to cell cycle arrest. They also activate DNA repair pathways.
Halting the Cell Cycle to Prevent Mutations
If the S phase checkpoint detects significant DNA damage or replication stress, it triggers a halt in cell cycle progression. This arrest provides the cell with an opportunity to repair the damage before it is copied and passed on to daughter cells.
By halting the cell cycle, these checkpoints prevent the propagation of mutations. Mutations can have detrimental consequences for the cell and organism. If repair is not possible, the checkpoint may trigger apoptosis, or programmed cell death, to eliminate the damaged cell.
This is a last-resort mechanism to prevent the damaged cell from dividing and potentially causing cancer. The careful balance between cell cycle progression and checkpoint control is essential for maintaining genomic stability and preventing disease.
Consequences of Errors in S Phase: Mutations and Cancer Development
The dance of DNA replication within the dynamically remodeled chromatin landscape is a feat of biological engineering. However, even with the best machinery, errors can arise. To safeguard the integrity of the genome, cells employ a sophisticated surveillance system.
As we've discussed, S phase is a period of intense activity where the entire genome is duplicated. But what happens when things go wrong? The consequences of errors during this critical phase can be far-reaching, potentially leading to mutations and contributing to the development of cancer.
The Mutagenic Potential of Replication Errors
DNA replication, despite its remarkable accuracy, is not infallible. Errors can and do occur.
These errors can range from simple base-pair mismatches to more complex insertions, deletions, or even structural rearrangements of the DNA.
If left uncorrected, these replication errors become permanent mutations, heritable changes in the DNA sequence. These mutations are then passed on to daughter cells.
The frequency of these errors is normally quite low thanks to proofreading mechanisms of DNA polymerases and the DNA repair systems, but these mechanisms aren't perfect. When errors do persist, the results can be devastating.
From Mutation to Malignancy: The Path to Cancer
Not all mutations are created equal. Some are silent, having no discernible effect on cellular function. Others, however, can disrupt critical cellular processes.
When mutations accumulate in genes that control cell growth, division, or DNA repair, the consequences can be dire.
These mutations can lead to uncontrolled cell proliferation, a hallmark of cancer.
For example, mutations in tumor suppressor genes can disable their ability to regulate cell division, while mutations in proto-oncogenes can convert them into oncogenes, driving excessive cell growth.
The accumulation of such mutations over time can gradually transform a normal cell into a cancerous one.
The Role of DNA Repair: A Cellular Safety Net
Fortunately, cells are not defenseless against replication errors. A complex network of DNA repair mechanisms constantly scans the genome. They identify and correct damage, including errors made during replication.
These repair pathways employ a variety of strategies, from directly reversing the damage to excising and replacing the affected DNA sequence.
These DNA repair mechanisms are crucial for maintaining genomic stability and preventing the accumulation of mutations.
However, the efficiency of DNA repair can be compromised by various factors, including aging, exposure to environmental toxins, and inherited genetic defects.
When DNA repair fails to keep pace with the rate of DNA damage, the risk of mutation and cancer development increases significantly.
S Phase in the Context of Cell Division
We've explored the intricacies of S phase, from the molecular machinery involved in DNA replication to the consequences of errors that may arise during this critical process. It's crucial now to zoom out and view S phase within the broader context of the cell cycle, understanding how it fits into the overall sequence of events that lead to cell division.
Preparing the Stage: S Phase and the Orchestration of Cell Division
S phase isn't an isolated event. It's a meticulously timed and regulated step that prepares the cell for the grand finale: cell division.
The successful completion of S phase, with the accurate duplication of the entire genome, is a prerequisite for cell division to proceed. Without a complete and accurate copy of the DNA, the resulting daughter cells would be genetically incomplete or flawed.
S phase essentially lays the foundation for the equal distribution of genetic material to the daughter cells during mitosis or meiosis. It's the point of no return – a commitment to division.
This commitment has ramifications for cell fate, requiring robust checkpoint mechanisms to ensure that DNA replication is completed before the cell moves into subsequent phases.
The G1 Phase: Growth and Preparation
Before a cell embarks on the journey of DNA replication in S phase, it typically resides in the G1 phase.
G1 (Gap 1) is a period of growth, metabolism, and preparation. During this phase, the cell increases in size, synthesizes proteins and organelles, and accumulates the necessary resources for DNA replication.
The decision to enter S phase is a critical one, and it's influenced by a variety of factors, including growth signals, nutrient availability, and the overall health of the cell.
Cells only progress from G1 to S if conditions are favorable. This transition is governed by checkpoints, which act as quality control mechanisms, ensuring that the cell is ready to replicate its DNA.
The G2 Phase: Final Checks and Balances
Following S phase, the cell enters G2 (Gap 2). This phase serves as a bridge between DNA replication and mitosis (or meiosis).
G2 is another period of growth and preparation, but it's primarily focused on ensuring that DNA replication has been completed accurately and that the cell is ready for cell division.
The cell checks for any DNA damage or errors that may have occurred during S phase and initiates repair mechanisms if necessary.
G2 phase provides a final opportunity to correct any mistakes before the cell commits to cell division. It's the last line of defense against the propagation of mutations.
The Interplay of Phases: A Harmonious Cycle
G1, S, and G2 phases are collectively known as interphase.
Interphase is a period of intense activity and preparation, setting the stage for the dramatic events of cell division.
The transitions between these phases are tightly regulated by cell cycle checkpoints, which monitor various aspects of cellular health and function.
These checkpoints ensure that each phase is completed successfully before the cell progresses to the next, preventing the propagation of errors and maintaining genetic stability.
The seamless coordination of G1, S, and G2 phases is essential for the proper functioning of cells and the overall health of an organism. It's a testament to the intricate and elegant mechanisms that govern cellular life.
Video: Unlock S Phase: The Secret To Perfect Cell Division (Explained)
FAQs: Understanding the S Phase of Cell Division
Here are some frequently asked questions to help you better understand the S phase and its importance in cell division.
What exactly happens during the S phase?
The S phase, short for synthesis phase, is the stage in the cell cycle where DNA replication occurs. During this phase, the cell duplicates its entire genome, ensuring that each daughter cell receives a complete and identical copy of the genetic material after cell division. Think of it as making a perfect copy of the instruction manual before splitting it up.
Why is the S phase so critical for successful cell division?
The S phase is absolutely vital. Without accurate DNA replication during the S phase, daughter cells would end up with incomplete or damaged genetic information. This can lead to mutations, cell death, or uncontrolled cell growth, which can contribute to diseases like cancer.
How does the cell ensure that DNA replication during the S phase is accurate?
Cells have sophisticated mechanisms to ensure accuracy during the S phase. These include DNA polymerases, which proofread and correct errors as they replicate DNA. Additionally, there are checkpoints that monitor DNA replication and halt cell cycle progression if problems are detected, giving the cell time to repair any damage before continuing.
What happens if something goes wrong during the S phase?
If errors occur during the S phase and are not repaired, the cell cycle may be arrested, or the cell may initiate programmed cell death (apoptosis) to prevent the propagation of damaged DNA. However, if these mechanisms fail, cells with damaged DNA can continue to divide, potentially leading to genetic instability and cancer development.