Phosphodiester Bond: DNA/RNA's Unsung Hero Explained
Hey there, future bio-whizzes! Ever wondered what holds the secrets of life together? It all boils down to something super tiny but incredibly powerful: the phosphodiester bond. Think of it as the molecular glue that makes up the backbone of both DNA, the famous double helix discovered by Watson and Crick, and its cousin, RNA, which is vital in protein synthesis. These phosphodiester bonds link individual nucleotides together, creating long chains that store and transmit genetic information. Scientists at institutions like the National Institutes of Health (NIH) study these bonds to better understand how genetic information is copied and passed on. With the help of advanced techniques like X-ray crystallography, we can even visualize these bonds, unlocking the secrets of heredity and paving the way for incredible advances in medicine and biotechnology!
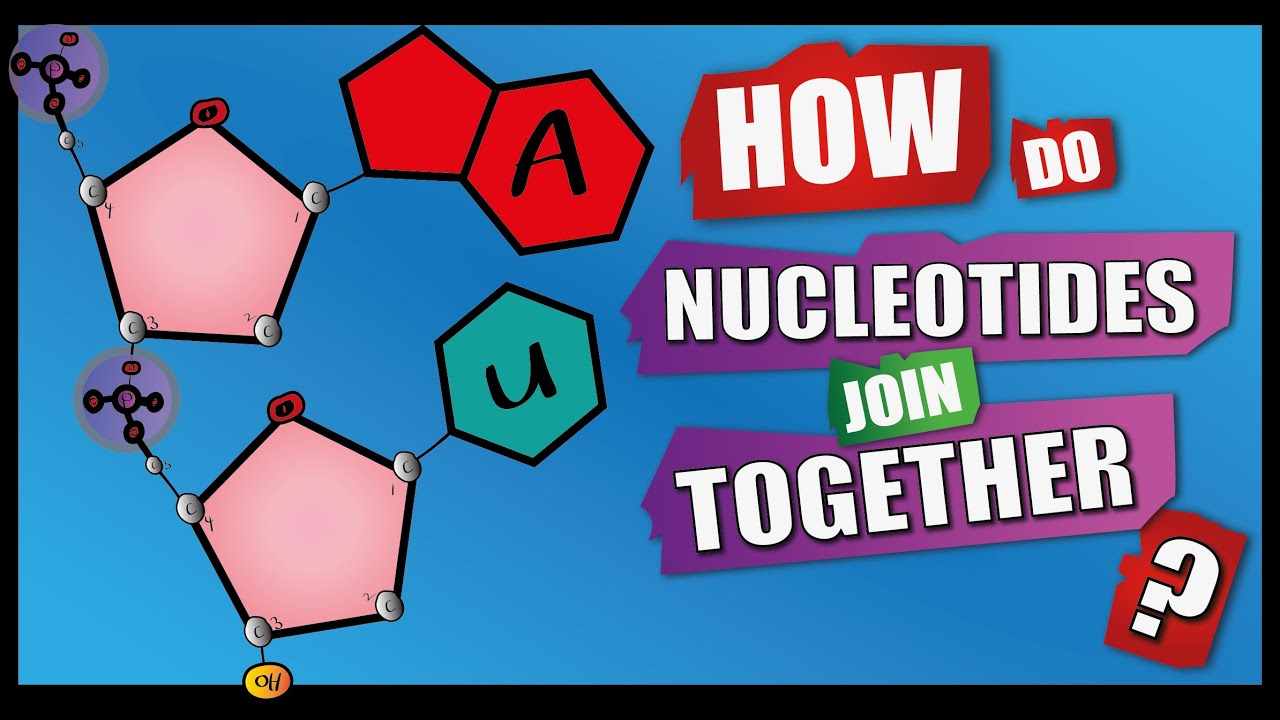
Image taken from the YouTube channel Learnbiologynet , from the video titled How Do Nucleotides Join Together? | A Level Biology | Phosphodiester Bonds | Condensation reactions .
Decoding Life: DNA, RNA, and the Phosphodiester Bond
Ever wondered how life manages to pass on its secrets, its very blueprint, from one generation to the next? The answer lies within the elegant structures of DNA and RNA, and at the heart of these molecules is the phosphodiester bond.
Let’s dive into these amazing molecules and see why these bonds are absolutely essential for life as we know it!
DNA and RNA: The Dynamic Duo of Genetics
DNA, or deoxyribonucleic acid, is the primary carrier of genetic information in most organisms. Think of it as the master blueprint, containing all the instructions needed to build and maintain a living thing. Its famous double helix structure isn't just aesthetically pleasing; it’s critical for its stability and function!
RNA, or ribonucleic acid, is a versatile molecule with a variety of roles. It's often seen as DNA’s hardworking assistant, involved in everything from protein synthesis (mRNA) to gene regulation (various non-coding RNAs).
While DNA resides primarily in the nucleus, RNA is more adventurous, moving around the cell to carry out its diverse tasks.
Nucleotides: The Building Blocks
Both DNA and RNA are polymers, meaning they are long chains of repeating units called nucleotides. Each nucleotide has three parts:
- A sugar molecule (deoxyribose in DNA, ribose in RNA)
- A phosphate group
- A nitrogenous base (adenine, guanine, cytosine, and either thymine in DNA or uracil in RNA).
It’s the sequence of these bases that encodes genetic information!
Think of nucleotides as Lego bricks that can be arranged in specific sequences to create complex structures. These sequences determine everything from eye color to enzyme function.
The Key Players: Phosphate, 3'-OH, and 5'-Phosphate
Now, let’s get to the heart of the matter: the phosphodiester bond. This bond is what links nucleotides together to form the long strands of DNA and RNA.
- The phosphate group is the linchpin, connecting to both the 3'-OH group (hydroxyl group attached to the 3' carbon) of one nucleotide and the 5'-phosphate group (phosphate group attached to the 5' carbon) of the next.
This creates a strong and stable chain, a backbone that holds the genetic information together.
Imagine the phosphate group as a clasp, firmly holding two nucleotides together. This creates a continuous strand that can carry an enormous amount of information.
Without these essential bonds, DNA and RNA wouldn't be able to form stable chains! Consequently, the genetic information within them wouldn’t be stored or passed on.
The phosphodiester bond is fundamental to life's processes, making it an unsung hero!
Building Blocks of Heredity: The Formation of Phosphodiester Bonds
Having explored the basic structure of DNA and RNA, it's time to journey deeper into the very act of creation – the formation of phosphodiester bonds. These aren't just static links; they are the dynamic bridges that build the ladder of life, allowing nucleotides to assemble into the long, information-rich strands of DNA and RNA that dictate our very being. How does this polymerization magic happen, and who are the key players in this molecular construction game? Let's find out!
The Polymerization Process: Stringing Together the Nucleotide Beads
Imagine stringing beads together to form a necklace. In the world of molecular biology, nucleotides are the beads, and the necklace is a strand of DNA or RNA. But instead of thread, we have the phosphodiester bond.
So how do nucleotides join?
It's a beautiful dance between the phosphate group on the 5' carbon of one nucleotide and the hydroxyl (-OH) group on the 3' carbon of another. This forms a strong covalent bond, releasing a water molecule in the process – a classic dehydration reaction.
Think of it like LEGO bricks clicking together!
This process repeats again and again, adding nucleotide after nucleotide, extending the chain to potentially millions or even billions of units long.
The result?
A single, incredibly long, and information-packed molecule, ready to carry the genetic code.
DNA and RNA Polymerases: The Master Builders
While the chemistry of phosphodiester bond formation is fascinating, it wouldn't happen with any degree of accuracy or speed without the help of specialized enzymes: DNA polymerases and RNA polymerases.
DNA Polymerases: The DNA Replication Experts
DNA polymerases are the workhorses of DNA replication, faithfully copying existing DNA strands to create new ones. They grab free nucleotides floating around the nucleus and, guided by the existing template strand, link them together via phosphodiester bonds.
But DNA polymerases aren’t just mindless robots!
They also have a built-in proofreading mechanism, correcting any errors they might make along the way. This ensures that the newly synthesized DNA strand is an almost perfect copy of the original.
RNA Polymerases: The Transcription Maestros
RNA polymerases, on the other hand, are responsible for transcription – creating RNA copies from a DNA template. They recognize specific sequences on the DNA, called promoters, that signal the start of a gene.
Once locked on, RNA polymerase unwinds a short stretch of DNA and begins assembling an RNA molecule, once again using phosphodiester bonds to link the RNA nucleotides together.
The amazing thing?
Different RNA polymerases exist, each tasked with transcribing different types of RNA, such as messenger RNA (mRNA), transfer RNA (tRNA), and ribosomal RNA (rRNA).
Transcription, Replication, and the Phosphodiester Bond
Ultimately, both transcription and replication rely entirely on the precise formation of phosphodiester bonds for accurate DNA and RNA copying. Without these bonds, the genetic information encoded in DNA would be impossible to access, transmit, or use.
Think about it.
- Replication: Ensures that each new cell receives a complete and accurate copy of the genome, made possible by phosphodiester bond formation.
- Transcription: Allows the information encoded in DNA to be used to synthesize proteins, the workhorses of the cell, also driven by phosphodiester bond formation.
So, the next time you think about heredity, remember the unsung hero: the phosphodiester bond, diligently linking nucleotides together, creating the very fabric of life!
Breaking and Remaking: Hydrolysis, Restriction Enzymes, and Ligases
Having explored the basic structure of DNA and RNA, it's time to journey deeper into the very act of creation – the formation of phosphodiester bonds. These aren't just static links; they are the dynamic bridges that build the ladder of life, allowing nucleotides to assemble into the long chains of genetic information. But what happens when those bonds need to be broken, or when DNA needs to be rearranged? That's where hydrolysis, restriction enzymes, and ligases enter the stage as molecular sculptors.
Hydrolysis: Water as the Wrecker Ball
Think of hydrolysis as the undo button in the story of life. It's a simple yet powerful reaction: the breaking of a chemical bond through the addition of water.
Specifically, a water molecule attacks the phosphodiester bond, cleaving it into two separate pieces. This process is crucial for recycling nucleotides and for degrading damaged DNA or RNA.
While hydrolysis can occur spontaneously, it's incredibly slow under physiological conditions. This is why we don't spontaneously dissolve! Instead, cells employ enzymes called nucleases to speed up the process.
These nucleases are like molecular demolition crews, precisely targeting phosphodiester bonds to break down nucleic acids when needed. It's a controlled deconstruction, ensuring that the building blocks of life can be reused or eliminated.
Restriction Enzymes: The Molecular Scissors
Now, let's talk about the real artists of DNA manipulation: restriction enzymes.
These amazing proteins, also known as restriction endonucleases, are bacterial in origin and act as a defense mechanism against viral infections.
They work by recognizing specific DNA sequences, called restriction sites, and cutting the DNA at or near those sites. Imagine them as molecular scissors, precisely snipping DNA at predetermined locations.
How Restriction Enzymes Work
Each restriction enzyme recognizes a unique DNA sequence, typically 4 to 8 base pairs long. These sequences are often palindromic, meaning they read the same forward on one strand as backward on the complementary strand.
Once the enzyme finds its target sequence, it cuts the phosphodiester bond, creating either "sticky ends" (overhanging single-stranded DNA) or "blunt ends" (clean cuts).
Applications of Restriction Enzymes
The discovery of restriction enzymes revolutionized molecular biology. They are indispensable tools for:
- DNA cloning: Creating recombinant DNA molecules by inserting a gene of interest into a plasmid vector.
- Genetic engineering: Modifying genes or genomes to introduce new traits or correct genetic defects.
- DNA fingerprinting: Analyzing DNA fragments to identify individuals based on their unique genetic profiles.
Ligases: The Molecular Glue
What happens after you've cut DNA with restriction enzymes? You need something to stick the pieces back together, right?
Enter ligases, the molecular glue of the biological world. These enzymes catalyze the formation of phosphodiester bonds, essentially stitching DNA fragments together.
How Ligases Work
Ligases use ATP (adenosine triphosphate) or NAD+ (nicotinamide adenine dinucleotide) as a source of energy to create a phosphodiester bond between the 3'-OH group of one nucleotide and the 5'-phosphate group of another.
They are essential for DNA replication, DNA repair, and, of course, genetic engineering.
Applications of Ligases
Ligases are the perfect partner to restriction enzymes, enabling scientists to:
- Create recombinant DNA: Join DNA fragments from different sources to create new combinations of genes.
- Repair DNA damage: Seal breaks in the DNA backbone to maintain the integrity of the genome.
- Amplify DNA: Close circular plasmids.
In short, ligases are the unsung heroes that maintain the structural integrity of DNA and enable countless molecular manipulations.
Molecular Toolkit: Applications Utilizing Phosphodiester Bonds
Having explored the basic structure of DNA and RNA, it's time to journey deeper into the very act of creation – the formation of phosphodiester bonds. These aren't just static links; they are the dynamic bridges that build the ladder of life, allowing nucleotides to assemble into the long, information-packed strands we call genetic material. But the real magic happens when we learn to manipulate these bonds. That's where our molecular toolkit comes in!
These tools, relying heavily on our understanding of phosphodiester bonds, have revolutionized biology. Let's dive into some key techniques.
Oligonucleotide Synthesis: Building Blocks on Demand
Imagine being able to order custom DNA sequences, designed to do exactly what you want. That's the power of oligonucleotide synthesis!
Oligonucleotides, short single-stranded DNA or RNA molecules, are synthesized chemically, nucleotide by nucleotide.
This precise control allows us to create primers for PCR, probes for hybridization, and even therapeutic agents.
The creation of synthetic oligonucleotides relies fundamentally on the controlled formation of phosphodiester bonds.
Think of it like this: each nucleotide is carefully added to the growing chain, forming a new phosphodiester bond with the previous nucleotide, all directed by automated machines with astonishing accuracy!
DNA Sequencing and RNA-Seq: Reading the Book of Life
DNA sequencing allows us to read the genetic code. Techniques like Sanger sequencing and next-generation sequencing (NGS) break DNA into fragments, amplify them, and then determine the order of nucleotides. RNA-Seq, similarly, sequences RNA molecules, giving us a snapshot of gene expression.
Both DNA sequencing and RNA-Seq rely on enzymes that interact with phosphodiester bonds.
Sequencing involves synthesizing a complementary strand of DNA, one nucleotide at a time. Identifying which nucleotide is added at each step reveals the original sequence.
The manipulation and detection of these phosphodiester-linked nucleotides is central to modern genomics.
PCR: Amplifying the Signal
Polymerase Chain Reaction (PCR) is a game-changer, allowing us to make millions of copies of a specific DNA sequence.
The process involves cycles of heating and cooling, driving denaturation, annealing, and extension.
Denaturation separates the DNA strands.
Annealing allows primers (those synthetic oligonucleotides we talked about earlier!) to bind to the target sequence.
Extension uses a DNA polymerase to extend the primers, creating new DNA strands.
Each cycle doubles the amount of DNA, leading to exponential amplification.
Because DNA polymerase builds that strand by – you guessed it – forming phosphodiester bonds! PCR is an indispensable technique in diagnostics, forensics, and research.
Gel Electrophoresis: Sorting DNA by Size
Gel electrophoresis is a simple yet powerful technique for separating DNA fragments based on size. DNA, being negatively charged due to the phosphate groups in its backbone (phosphodiester bonds, remember!), migrates through a gel matrix towards a positive electrode.
Smaller fragments move faster than larger fragments, allowing us to separate DNA molecules by size.
Gel electrophoresis is used to visualize DNA fragments after PCR, confirm the size of synthesized oligonucleotides, and analyze DNA digests created by restriction enzymes.
It's a fundamental tool for any molecular biologist, providing a quick and easy way to assess the size and purity of DNA samples. The phosphate backbone is key.
The Big Picture: Phosphodiester Bonds, Genetic Code, and Molecular Stability
Having explored the basic structure of DNA and RNA, it's time to zoom out and see the grand scheme of things. How do those tiny phosphodiester bonds contribute to the flow of information that defines life? They're not just there to connect nucleotides; they are crucial to the integrity of the genetic code and the central dogma itself.
Phosphodiester Bonds: The Backbone of the Genetic Code
Imagine DNA as a meticulously crafted book of life. Each nucleotide is a letter, and the phosphodiester bonds are the glue that binds those letters into meaningful words (genes!).
Without those bonds, the entire genetic code would fall apart into a meaningless jumble.
They provide the structural scaffolding needed for the precise order of bases (A, T, C, G) that dictates our traits. It's this precise sequence that encodes for proteins.
The Central Dogma: Phosphodiester Bonds in Action
The central dogma of molecular biology is DNA → RNA → Protein. It describes the flow of genetic information.
Phosphodiester bonds are actively involved in this whole process!
Transcription: Building RNA
During transcription, RNA polymerase creates an RNA copy of a DNA sequence. This process is entirely dependent on the formation of phosphodiester bonds between RNA nucleotides, guided by the DNA template.
Think of RNA polymerase as a skilled builder, using phosphodiester bonds as mortar. These bonds are the mortar that joins the RNA nucleotides into a growing RNA chain.
Translation: Using the Code
While translation primarily involves ribosomes and tRNA, it’s the integrity of the mRNA (built on phosphodiester bonds) that ensures the correct amino acid sequence is assembled.
A faulty mRNA transcript means a faulty protein, and that can have drastic consequences.
Molecular Stability: Phosphodiester Bonds and DNA's Longevity
DNA needs to stick around for the long haul, right? It has to survive replication, transcription, and all sorts of cellular stresses. This is where the hydrolytic stability of phosphodiester bonds comes into play.
Why Hydrolytic Stability Matters
Phosphodiester bonds are relatively resistant to hydrolysis. Hydrolysis is the breaking of a chemical bond using water.
This inherent stability protects the genetic information from degrading too quickly. Can you imagine if our DNA just fell apart every few minutes?
Contributing Factors
The structure of the phosphodiester bond shields it from rapid breakdown in water. It helps to ensure genetic information remains intact over time, from one generation to the next.
This stability is essential for the reliable transmission of genetic information through cell divisions and across generations.
In other words, these bonds are the unsung heroes, quietly ensuring that the blueprint of life is preserved and accurately passed on.
Pioneers of the Code: Unveiling the Secrets of DNA and RNA
Having explored the pivotal role of phosphodiester bonds in maintaining the genetic code and facilitating the central dogma of molecular biology, it's important to acknowledge the giants upon whose shoulders we stand. These unsung heroes dedicated their lives to unraveling the molecular mechanisms of life. Let's dive into the brilliant minds that illuminated the path!
Specifically, we'll shine a spotlight on Arthur Kornberg and Severo Ochoa, two titans whose groundbreaking work with DNA and RNA polymerases revolutionized our understanding of replication and transcription.
Arthur Kornberg: The Father of DNA Polymerase
Arthur Kornberg, a name synonymous with DNA replication, gifted the world with one of the most pivotal enzymes in molecular biology: DNA polymerase I. His relentless pursuit of understanding how DNA duplicates itself earned him the Nobel Prize in Physiology or Medicine in 1959.
Kornberg's Breakthrough with DNA Polymerase I
Imagine trying to understand how a complex machine works without even knowing its fundamental components. That was the challenge Kornberg faced.
He meticulously isolated and characterized DNA polymerase I from E. coli, a bacterium that became a cornerstone of molecular biology.
His in vitro replication experiments, using purified enzymes and nucleotide building blocks, revealed the step-by-step mechanism of DNA synthesis.
This was a watershed moment, providing the first glimpse into how genetic information is faithfully copied and passed on to future generations.
Kornberg's work established that DNA polymerase I could indeed synthesize new DNA strands using an existing DNA template, laying the foundation for all subsequent research in DNA replication.
While DNA polymerase I isn't the main replicative polymerase in E. coli (that honor belongs to DNA polymerase III), Kornberg's discovery paved the way for the discovery and characterization of all polymerases.
He showed how it could be done!
The Significance of Kornberg's Discovery
Kornberg's discovery wasn't just an isolated finding; it opened the floodgates for understanding DNA replication in all organisms.
His work had immediate and profound implications for understanding how cells divide, how genetic mutations arise, and how genetic information is transmitted across generations.
Think about it: every time a cell divides, its entire genome must be accurately duplicated. Kornberg's work revealed the molecular machinery responsible for this incredible feat of biological engineering.
His research also spurred the development of numerous techniques used in modern molecular biology, including DNA sequencing and polymerase chain reaction (PCR). Without Kornberg's initial discoveries, these transformative technologies might never have existed.
Severo Ochoa: Decoding the Secrets of RNA Synthesis
While Kornberg focused on DNA, Severo Ochoa delved into the mysteries of RNA, another essential nucleic acid. He was awarded the Nobel Prize alongside Kornberg, also in 1959, for his groundbreaking work on the enzymatic synthesis of RNA.
Ochoa's Contribution to RNA Polymerase Understanding
Ochoa isolated and characterized polynucleotide phosphorylase, an enzyme that can synthesize RNA in vitro.
While initially thought to be the primary RNA polymerase, it was later discovered to function more prominently in RNA degradation.
Nevertheless, Ochoa's work was crucial in understanding the basic principles of RNA synthesis.
He demonstrated that RNA could be synthesized from nucleotide precursors, just as Kornberg had shown for DNA.
This discovery enabled researchers to produce synthetic RNA molecules, which were essential for deciphering the genetic code.
Deciphering the Genetic Code with Synthetic RNA
One of the most significant applications of Ochoa's work was its use in cracking the genetic code. By synthesizing RNA molecules with specific sequences, researchers could use these molecules to direct protein synthesis in vitro.
By observing which amino acids were incorporated into the resulting proteins, they could determine which RNA sequences corresponded to which amino acids.
This research culminated in the complete deciphering of the genetic code, a monumental achievement in molecular biology. Without Ochoa's work, this critical step in understanding life's blueprint would have been significantly delayed.
Legacies Etched in Molecular Biology
The contributions of Kornberg and Ochoa extend far beyond their initial discoveries.
Their work inspired generations of scientists to pursue careers in molecular biology and genetics.
Their meticulous experimental approaches and unwavering dedication to scientific rigor set a high standard for research in the field.
The tools and techniques developed as a result of their work are now used in countless laboratories around the world, driving innovation in medicine, biotechnology, and agriculture.
The foundations for much of modern molecular biology lie in their pioneering investigations into the enzymatic synthesis of DNA and RNA. Let's not forget those who built the road ahead!
Video: Phosphodiester Bond: DNA/RNA's Unsung Hero Explained
FAQs: Phosphodiester Bonds
What exactly does a phosphodiester bond do in DNA or RNA?
A phosphodiester bond connects nucleotides in DNA and RNA, forming the sugar-phosphate backbone. It links the 3' carbon atom of one sugar molecule to the 5' carbon atom of the next, creating a chain. This is how genetic information is linearly strung together.
Why is the phosphodiester bond considered an "unsung hero"?
The phosphodiester bond is crucial because it's exceptionally strong and stable under physiological conditions. This stability is essential for the long-term storage and reliable transmission of genetic information. Without this bond's integrity, DNA and RNA would degrade quickly, preventing inheritance.
How does the formation of a phosphodiester bond create the DNA/RNA backbone?
The phosphodiester bond forms through a dehydration reaction, where a water molecule is removed. The phosphate group connects the 3' hydroxyl of one nucleotide's sugar to the 5' hydroxyl of the next nucleotide's sugar. Repeating this process creates the continuous sugar-phosphate backbone linked by phosphodiester bonds.
Can the phosphodiester bond be broken, and if so, how?
Yes, the phosphodiester bond can be broken, usually through enzymatic hydrolysis. Enzymes called nucleases can cleave the bond. Also, chemicals or radiation can disrupt the phosphodiester bond, leading to DNA or RNA damage.
So, the next time you're thinking about DNA or RNA, remember the unsung hero: the phosphodiester bond. It might not be as famous as the double helix, but it's the strong and silent type, holding the very blueprint of life together. Pretty cool, right?