Unlock the Secret: Lock and Key Model Explained! (60 Char)
The enzyme, a crucial biological catalyst, exhibits remarkable specificity, a characteristic deeply rooted in the lock and key model. This model, first proposed by Emil Fischer, elegantly illustrates the interaction between an enzyme's active site and its specific substrate. Consequently, understanding the lock and key model becomes fundamental when investigating the intricacies of biochemical reactions within cellular environments.
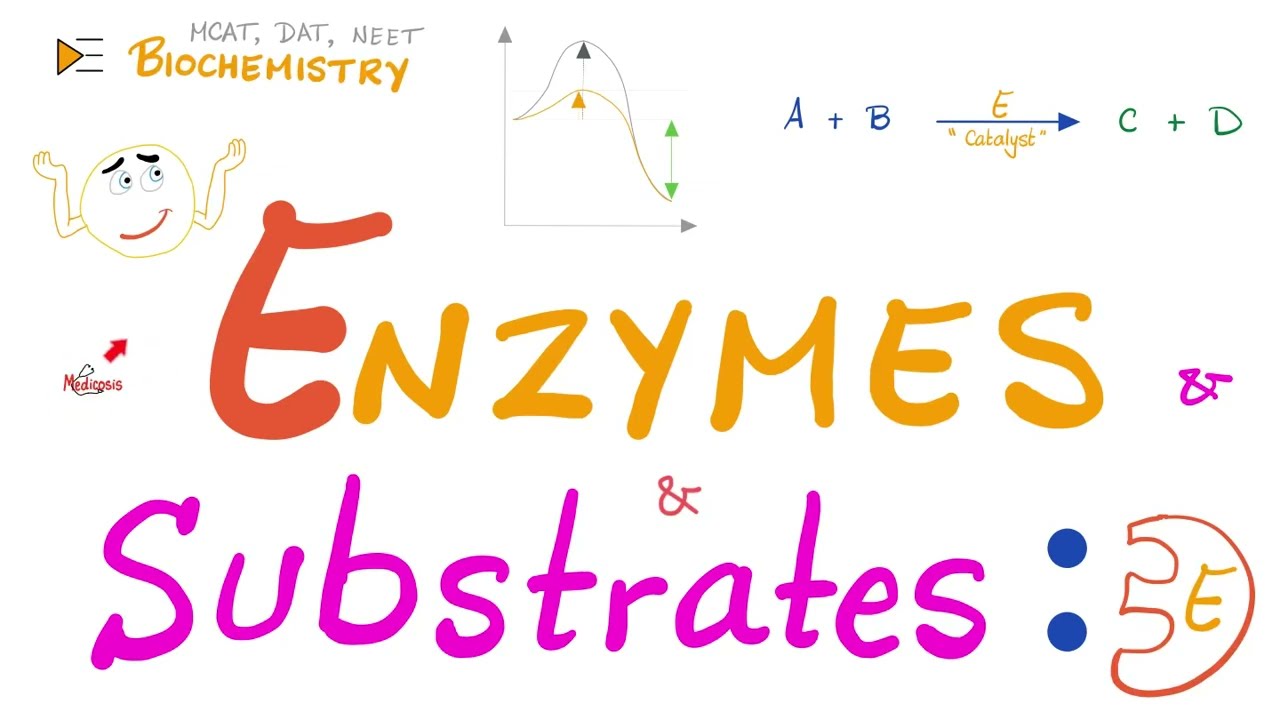
Image taken from the YouTube channel Medicosis Perfectionalis , from the video titled Enzyme Activity - Enzyme and Substrate - Key and Lock Theory vs. Induced Fit Model - Biochemistry 🧪 .
Imagine holding a complex lock. Only one key, meticulously crafted with a unique pattern, can unlock it. This simple analogy perfectly illustrates the elegant and fundamental concept of the lock and key model in biochemistry.
At the heart of countless biological processes lies the remarkable ability of enzymes to selectively interact with specific molecules. Just as a key fits a particular lock, an enzyme binds to a specific substrate, initiating a cascade of events crucial for life.
The Lock and Key Model: A Primer
The lock and key model describes the highly specific interaction between an enzyme and its substrate. This model proposes that the active site of an enzyme has a rigid shape that is perfectly complementary to the shape of its substrate.
This precise fit ensures that the enzyme only binds to its intended target, preventing unwanted reactions and maintaining the integrity of cellular processes. This specificity is not merely a matter of shape; it also involves specific chemical properties and charge distributions that facilitate optimal binding.
The lock and key model is significant in biochemistry and biology for several reasons:
-
It provides a framework for understanding enzyme specificity, a crucial aspect of biological reactions.
-
It helps explain how enzymes can catalyze reactions with high efficiency.
-
It highlights the importance of molecular shape and structure in biological interactions.
Purpose of This Article
This article will provide a comprehensive exploration of the lock and key model, delving into its historical context, key components, limitations, and real-world applications. We will also discuss the role of specificity in enzymatic reactions.
Our aim is to offer a clear and insightful understanding of this foundational concept in biochemistry, equipping you with the knowledge to appreciate its significance in the intricate machinery of life.
The Core Concept: Enzyme-Substrate Specificity Explained
The beauty of the lock and key model lies in its simplicity, yet it underpins a fundamental aspect of life itself: the highly specific interaction between an enzyme and its substrate. Like a precisely crafted key designed for a single lock, an enzyme is tailored to bind with a particular molecule, the substrate, and no other.
This exquisite selectivity is not accidental; it's the cornerstone of efficient and controlled biochemical reactions. Without it, cellular processes would descend into chaotic, unproductive interactions.
The Active Site: Where the Magic Happens
At the heart of this specificity lies the active site, a region within the enzyme with a unique three-dimensional structure. This pocket or cleft is meticulously shaped to perfectly accommodate the substrate, much like a glove fits a hand.
The active site isn't merely a physical space; it's a carefully designed microenvironment with specific chemical properties.
Amino acid residues lining the active site play crucial roles, forming temporary bonds with the substrate through interactions like hydrogen bonding, hydrophobic interactions, and electrostatic forces.
These interactions ensure that the substrate is held securely and precisely oriented within the active site, poised for the catalytic reaction.
Complementarity: Shape and Chemical Properties
The complementary nature of the enzyme's active site and the substrate is paramount. This complementarity extends beyond just the overall shape; it also encompasses the distribution of charges, hydrophobic regions, and other chemical features.
The substrate must not only fit into the active site but also possess the correct chemical properties to interact favorably with the amino acid residues within.
Think of it as a key not only having the right shape but also being made of the right material to interact with the tumblers in the lock.
Specificity: The Key to Biological Order
The specificity of enzymatic reactions is what allows cells to carry out thousands of different biochemical processes simultaneously and without interference.
Each enzyme is designed to catalyze a specific reaction with a specific substrate, ensuring that metabolic pathways proceed in an orderly and controlled manner.
This specificity prevents enzymes from indiscriminately binding to other molecules and causing unwanted reactions, which could disrupt cellular function and lead to detrimental consequences.
Enzyme specificity is not just a matter of efficiency; it's a matter of survival. It's a fundamental principle that enables life to exist in its complex and organized form.
The precision of enzyme-substrate interactions, as we’ve seen, is a cornerstone of biochemistry. But understanding this mechanism wasn't instantaneous. It required the insight of pioneering scientists who laid the groundwork for our modern understanding of enzymatic processes.
A Glimpse into History: Emil Fischer's Vision
The story of the lock and key model is inextricably linked to the work of Emil Fischer, a towering figure in the history of organic chemistry and biochemistry. His meticulous research and insightful thinking paved the way for our comprehension of enzyme specificity.
Introducing Emil Fischer: A Pioneer in Stereochemistry
Emil Fischer (1852-1919) was a German chemist renowned for his work on sugars, purines, and enzymes. His research wasn't limited to simply observing reactions. He sought to understand the underlying structural principles that governed these interactions.
Fischer's work on carbohydrates, particularly his elucidation of the structure of sugars, earned him the Nobel Prize in Chemistry in 1902. This accomplishment laid the foundation for his later investigations into enzyme action.
Fischer's Postulation: The Lock and Key Analogy
In 1894, Fischer proposed the lock and key analogy to explain the remarkable specificity observed in enzyme-substrate interactions. He hypothesized that an enzyme's active site possesses a rigid shape that is perfectly complementary to the shape of its substrate.
Just as a specific key is required to unlock a particular lock, only a substrate with the correct three-dimensional structure could bind to a given enzyme and undergo catalysis.
This rigid complementarity was the key insight of Fischer's model. It provided a tangible and readily understandable explanation for the selective nature of enzymatic reactions.
Revolutionizing the Understanding of Biochemical Reactions
Fischer's lock and key model represented a paradigm shift in the understanding of biochemical reactions. Before his work, the prevailing view was that enzymes were simply general catalysts, capable of acting on a wide range of substrates.
Fischer's model, however, underscored the importance of molecular structure and shape in determining enzyme specificity. It highlighted the idea that enzymes are highly specialized catalysts, each designed to interact with a particular substrate.
By emphasizing the importance of stereochemistry and the three-dimensional structure of molecules, Fischer's model provided a framework for understanding how enzymes could achieve such high levels of selectivity.
His vision revolutionized the field of biochemistry, setting the stage for future investigations into the intricacies of enzyme structure and function. While the model has been refined over time, its core principle of complementary shape remains a cornerstone of our understanding of enzyme-substrate interactions.
The rigid complementarity was the key insight of Fischer's model. It provided a simple yet powerful explanation for why enzymes exhibit such exquisite selectivity in their interactions. Now, let's dissect the components that make this lock and key mechanism work: the enzymes, the substrates, and the all-important active site.
Key Players: Enzymes, Substrates, and the Active Site
The lock and key model, elegant in its simplicity, hinges on three critical elements: enzymes, substrates, and the active site. Understanding the specific roles each plays is crucial to appreciating the overall mechanism and its biological implications.
Enzymes: The Biological Catalysts
Enzymes are, quite simply, the workhorses of biological systems. As biological catalysts, they accelerate chemical reactions within cells, reactions that would otherwise proceed far too slowly to sustain life.
Without enzymes, metabolic processes like digestion, respiration, and muscle contraction would grind to a halt. Enzymes are protein molecules with a specific three-dimensional structure, which is critical for their function.
Their catalytic power stems from their ability to lower the activation energy of a reaction, effectively providing an easier pathway for the reaction to occur. This reduction in activation energy can speed up reactions by factors of millions or even billions.
Enzymes are highly specific, meaning each enzyme typically catalyzes only one particular reaction or a set of very similar reactions. This specificity is a direct consequence of the lock and key principle.
Substrates: The Molecules Acted Upon
The substrate is the molecule upon which an enzyme acts. It's the substance that is chemically modified in the enzymatic reaction.
Substrates bind to the enzyme at a specific location called the active site, initiating the catalytic process. The nature of the substrate dictates which enzyme it can interact with, ensuring that the correct reactions occur in the proper sequence within the cell.
The concentration of the substrate often influences the rate of the enzymatic reaction. At low substrate concentrations, the reaction rate typically increases as more substrate becomes available. However, at high substrate concentrations, the enzyme can become saturated, and the reaction rate plateaus.
The Active Site: The Heart of the Interaction
The active site is a region on the enzyme with a specific three-dimensional structure that is complementary to the structure of the substrate. It's where the magic happens, where substrate binding and catalysis take place.
Structure of the Active Site
The active site isn't simply a groove or pocket on the enzyme's surface. It's a precisely shaped microenvironment, often formed by amino acid side chains that are brought together by the enzyme's folding pattern.
These amino acid residues can participate directly in the catalytic reaction. They may also help to stabilize the transition state, the unstable intermediate formed during the reaction.
Binding and Catalysis
The shape and chemical properties of the active site are crucial for both binding the substrate and catalyzing the reaction. The complementary shape ensures that the substrate fits snugly into the active site, much like a key fitting into a lock.
Once the substrate is bound, the enzyme facilitates the chemical reaction, transforming the substrate into one or more products. After the reaction is complete, the products are released, and the enzyme is free to bind another substrate molecule and repeat the process. The precise arrangement of amino acids within the active site not only ensures substrate specificity but also provides the optimal conditions for catalysis to occur.
The specificity of the enzyme, the perfect fit of the substrate, and the architecture of the active site paint a beautiful picture of molecular recognition. However, this "lock and key" interaction, while fundamental, isn't set in stone. Several environmental factors can influence the efficiency and even the possibility of this interaction. These factors, often subtle, play a pivotal role in modulating enzymatic activity and, consequently, biological processes.
Factors Influencing the Lock and Key Model
The elegant simplicity of the lock and key model doesn't exist in a vacuum. External conditions and inherent molecular properties can significantly impact the binding and catalytic efficiency of enzymes. Understanding these influences is critical to fully appreciating the complexities of enzyme function.
Temperature: A Delicate Balance
Temperature exerts a profound influence on enzyme activity.
Enzymes, being proteins, are sensitive to temperature fluctuations.
As temperature increases, the rate of enzyme-catalyzed reactions generally rises, as molecules possess more kinetic energy, leading to more frequent collisions between enzyme and substrate.
However, this trend holds only up to a certain point. Beyond an optimal temperature, the enzyme's delicate three-dimensional structure begins to unravel, a process known as denaturation.
Denaturation disrupts the shape of the active site, rendering the enzyme incapable of binding to its substrate.
Think of it like trying to fit a key into a lock that has been warped by heat – the perfect fit is lost, and the mechanism fails.
pH Levels: Fine-Tuning the Active Site
pH, a measure of acidity or alkalinity, also plays a critical role in maintaining the integrity of the enzyme's active site.
Enzymes function optimally within a narrow pH range.
Changes in pH can alter the ionization state of amino acid residues within the active site, affecting their ability to interact with the substrate.
Extreme pH values can also lead to denaturation, similar to the effects of high temperature.
The optimal pH for an enzyme is often closely tied to the environment in which it naturally functions.
For instance, pepsin, an enzyme found in the stomach, functions best at a highly acidic pH, while enzymes in the small intestine typically prefer a more neutral pH.
Binding Affinity: The Strength of the Interaction
Binding affinity refers to the strength of the attraction between an enzyme and its substrate.
A high binding affinity means the enzyme and substrate bind tightly and remain associated for a longer period, facilitating catalysis.
Conversely, a low binding affinity indicates a weaker interaction, potentially hindering the reaction.
Several factors can affect binding affinity, including:
- The precise complementarity of the active site and substrate: The better the fit, the stronger the binding.
- The presence of cofactors or coenzymes: Some enzymes require these helper molecules to enhance substrate binding.
- Environmental conditions: Temperature and pH can also influence binding affinity by affecting the enzyme's structure.
Enzyme Inhibition: Blocking the Keyhole
Enzyme inhibitors are molecules that reduce or prevent enzyme activity.
They act by interfering with the enzyme-substrate interaction, effectively "blocking the keyhole" or altering the key itself.
Enzyme inhibition can be:
-
Reversible: The inhibitor binds non-covalently and can be removed, restoring enzyme activity.
-
Competitive inhibition: The inhibitor binds to the active site, directly competing with the substrate.
-
Non-competitive inhibition: The inhibitor binds to a different site on the enzyme, altering its shape and reducing its activity.
-
-
Irreversible: The inhibitor binds covalently to the enzyme, permanently disabling it.
Enzyme inhibitors are crucial in regulating metabolic pathways and serve as the basis for many drugs and pesticides.
Understanding the mechanisms of enzyme inhibition is vital in pharmacology and toxicology.
Beyond the Lock and Key: The Induced Fit Model
While the lock and key model provides a valuable foundation for understanding enzyme-substrate interactions, it presents a somewhat static and rigid view of the process. Nature, however, is rarely so inflexible. The reality of enzyme function is more dynamic, involving conformational changes and adaptable interactions. This is where the induced fit model comes into play, offering a more nuanced and accurate representation of enzymatic activity.
A Refinement of the Lock and Key
The induced fit model builds upon the original lock and key concept, acknowledging the fundamental principle of complementary shapes. However, it goes a step further by proposing that the active site of an enzyme is not perfectly pre-formed to fit the substrate. Instead, the enzyme's active site is more flexible, adopting its optimal shape only after the substrate binds.
Imagine a glove that slightly molds to the shape of your hand upon insertion. The glove and hand are initially somewhat compatible, but the fit becomes far more precise as they interact. This is analogous to the induced fit model, where the enzyme undergoes a conformational change to maximize its interaction with the substrate.
Conformational Changes: A Dynamic View
The induced fit model emphasizes the dynamic nature of enzyme-substrate interactions. When a substrate binds to the enzyme, it induces a change in the enzyme's three-dimensional structure. This change can involve the movement of amino acid side chains within the active site, bringing catalytic groups into the optimal position for the reaction to occur.
This flexibility is crucial for several reasons. It allows the enzyme to:
- Optimize its binding affinity for the substrate.
- Exclude water molecules from the active site, creating a more favorable environment for catalysis.
- Apply strain to the substrate, facilitating the breaking or formation of chemical bonds.
Lock and Key vs. Induced Fit: A Comparison
The key difference between the two models lies in the rigidity of the enzyme's active site.
The lock and key model proposes a pre-existing, perfectly complementary fit between the enzyme and substrate.
In contrast, the induced fit model suggests a more flexible interaction, where the enzyme's active site molds itself around the substrate.
Think of it this way:
- Lock and key: A specific key fits into only one specific lock, with no change in the lock's shape.
- Induced fit: A flexible lock adjusts its shape slightly to better accommodate a key, creating a tighter and more secure fit.
While the lock and key model is a useful starting point for understanding enzyme specificity, the induced fit model provides a more comprehensive and accurate depiction of the dynamic interplay between enzymes and substrates.
The Role of Proteins and Activation Energy
Proteins, as the building blocks of enzymes, are central to the induced fit model. The flexibility and conformational changes described above are possible because of the complex three-dimensional structure of proteins, which is held together by a network of weak interactions. These interactions can be readily rearranged to allow the enzyme to adapt to the substrate.
The induced fit model also has implications for activation energy, the energy required to initiate a chemical reaction. By optimizing its interaction with the substrate, the enzyme can stabilize the transition state, the high-energy intermediate formed during the reaction. This stabilization lowers the activation energy, making the reaction proceed more rapidly. The conformational changes are essential for reaching that transition state and pushing the reaction forward. Ultimately, lowering the activation energy allows reactions to occur at biologically relevant temperatures and time scales.
Of course, let's expand the "Real-World Applications: Examples of Lock and Key in Action" section into a comprehensive, analytical editorial section.
Real-World Applications: Examples of Lock and Key in Action
While the induced fit model offers a refined perspective, the lock and key model continues to provide a valuable framework for understanding numerous biological interactions. Its simplicity makes it particularly useful for illustrating the fundamental principles of enzyme-substrate and receptor-ligand specificity in various real-world scenarios. Let's delve into some concrete examples that showcase the enduring relevance of this model.
Metabolic Pathways: A Lock and Key Foundation
Many metabolic pathways rely on the precise interaction between enzymes and their substrates, perfectly illustrating the lock and key principle. Consider, for example, the role of lactase in the digestion of lactose, a sugar found in milk.
Lactase, the enzyme, possesses a specific active site that is structurally complementary to lactose, the substrate. This complementary fit allows lactase to bind to lactose, breaking it down into glucose and galactose, which are then absorbed into the bloodstream.
In individuals with lactose intolerance, lactase production is insufficient. The lactose molecules cannot be properly processed due to the lack of sufficient “key”, leading to digestive discomfort. This example clearly demonstrates the specificity inherent in the lock and key model.
Another compelling example can be found in the function of amylase. This enzyme, present in saliva and pancreatic fluids, initiates the breakdown of starch (a complex carbohydrate) into simpler sugars.
Amylase features an active site specifically designed to accommodate the structure of starch molecules. This precise fit enables amylase to efficiently hydrolyze the glycosidic bonds within starch, initiating the digestive process.
Receptor-Ligand Interactions: Cellular Communication
The lock and key model is equally applicable to understanding receptor-ligand interactions, which are fundamental to cell signaling and communication. Receptors, typically located on the cell surface, act as the "locks," while ligands, such as hormones or neurotransmitters, serve as the "keys."
The binding of a specific ligand to its corresponding receptor triggers a cascade of intracellular events, ultimately leading to a cellular response.
Insulin and Its Receptor
A classic example is the interaction between insulin and its receptor on cells. Insulin, a hormone produced by the pancreas, regulates blood glucose levels. It binds to the insulin receptor on target cells, such as liver and muscle cells.
The insulin receptor has a highly specific binding site that recognizes and binds only to insulin. Upon binding, the receptor undergoes a conformational change, initiating a signaling pathway that promotes glucose uptake from the bloodstream into the cell. This lowers blood sugar levels.
Neurotransmitters and Their Receptors
The nervous system relies heavily on receptor-ligand interactions for communication between neurons. Neurotransmitters, such as acetylcholine or serotonin, act as ligands, binding to specific receptors on the postsynaptic neuron.
For example, acetylcholine binds to acetylcholine receptors at the neuromuscular junction, triggering muscle contraction. Serotonin, on the other hand, binds to serotonin receptors in the brain, influencing mood, sleep, and appetite.
The specificity of these interactions ensures that the correct signal is transmitted, allowing for precise control of various physiological processes. These examples, from metabolic pathways to cell signaling, underscore the enduring importance of the lock and key model in understanding biological specificity.
Video: Unlock the Secret: Lock and Key Model Explained! (60 Char)
FAQs: Understanding the Lock and Key Model
Here are some common questions about the lock and key model and how it works.
What exactly is the lock and key model?
The lock and key model is a concept that explains how specific molecules, like enzymes and substrates, interact. It suggests that a substrate (the "key") fits perfectly into the active site of an enzyme (the "lock"), allowing a reaction to occur.
How is the lock and key model used in enzyme reactions?
Enzymes have a specific shape due to their amino acid sequence. The lock and key model illustrates that only a substrate with a complementary shape can bind to the enzyme's active site, forming a complex that facilitates the reaction.
Is the lock and key model a perfect analogy?
While helpful, the lock and key model is a simplification. The induced fit model provides a more accurate representation. It describes that the active site can slightly change its shape to better accommodate the substrate.
What happens after the reaction in the lock and key model?
After the enzyme catalyzes the reaction, the products are released. The enzyme, unchanged, can then bind to another substrate molecule. This cycle repeats, allowing the enzyme to catalyze numerous reactions based on the lock and key model's specificity.