Fraction of a Joule: Examples and Uses Explained
A fraction of a joule represents an exceedingly small quantity of energy, often encountered in scenarios where precision and minimal impact are paramount. Consider, for instance, the realm of microelectronics, where the operation of a single transistor, a fundamental building block in devices designed by Intel, may require energy on the order of a fraction of a joule. Similarly, in laser technology, specifically within laser-induced breakdown spectroscopy (LIBS) employed by organizations such as NASA for material analysis on Mars, the energy delivered in short pulses can be finely tuned to levels equivalent to a fraction of a joule to ablate material for study. Moreover, the field of biophysics frequently deals with energy transfers at the cellular level, where processes such as ion channel activation involve energy changes that constitute a fraction of a joule.
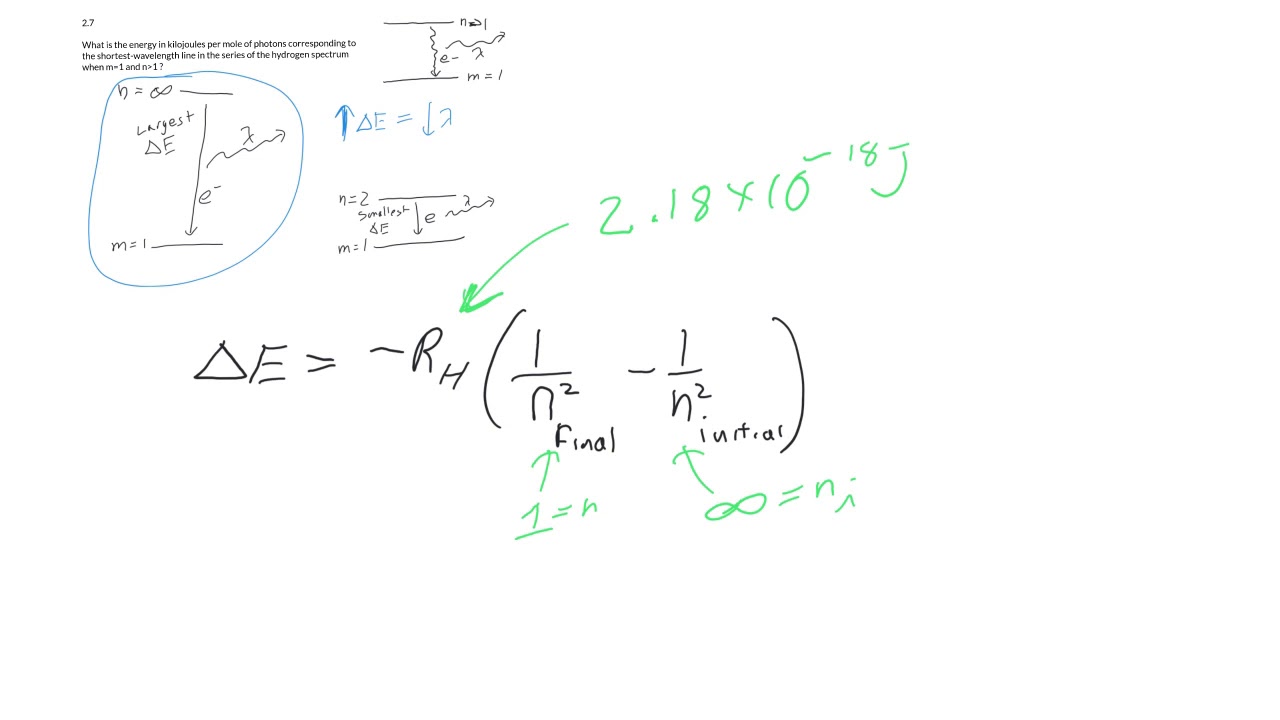
Image taken from the YouTube channel The Doctor Kellie , from the video titled 2 7 .
Unveiling the Power of the Joule
The Joule (J) stands as the bedrock upon which our understanding and quantification of energy are built. Within the International System of Units (SI), it reigns supreme as the fundamental unit of energy. It is the measure against which all other forms of energy—kinetic, potential, thermal, and electrical—are ultimately compared and expressed.
Defining Energy and the Joule
At its essence, energy is defined as the capacity to do work. It represents the potential to exert a force over a distance. The Joule, therefore, quantifies the amount of energy transferred when a force of one Newton is applied over a distance of one meter.
Mathematically, 1 J = 1 N⋅m = 1 kg⋅m²/s².
This seemingly simple definition belies the profound implications and far-reaching applications of the Joule across the scientific and engineering landscape.
The Ubiquitous Joule: From Physics to Engineering
The Joule is not confined to the theoretical realms of physics. Its influence permeates virtually every corner of science and engineering.
In physics, it is used to calculate kinetic energy, potential energy, and work done by forces.
In chemistry, it measures the energy released or absorbed during chemical reactions (enthalpy).
In electrical engineering, it quantifies electrical energy consumption and storage.
Mechanical engineering relies on the Joule to calculate the energy involved in mechanical processes like motion and deformation.
Why Understanding the Joule Matters
Understanding the Joule is not merely an academic exercise; it is a practical imperative.
It is the foundation upon which we design energy-efficient systems, analyze power consumption, and develop innovative technologies.
From optimizing the performance of microelectronic devices to assessing the energy efficiency of buildings, the Joule provides a common language and a standardized framework for quantifying and comparing energy usage.
In essence, grasping the significance of the Joule empowers us to make informed decisions about energy consumption and to contribute to a more sustainable future.
Deciphering Energy Measurement: Work, Heat, and Motion
[Unveiling the Power of the Joule The Joule (J) stands as the bedrock upon which our understanding and quantification of energy are built. Within the International System of Units (SI), it reigns supreme as the fundamental unit of energy. It is the measure against which all other forms of energy—kinetic, potential, thermal, and electrical—are ultima...]
Understanding the Joule requires a firm grasp on the core concepts it quantifies. Energy manifests in various forms, each with unique characteristics and mechanisms of transfer. Let's delve into these fundamental aspects of energy measurement—work, heat, kinetic energy, and potential energy—to build a robust foundation for understanding how Joules are used to describe the physical world.
Work: Energy Transfer Through Force
In physics, work is defined precisely as the transfer of energy that occurs when a force causes displacement. It's not simply about exertion; it's about a force acting over a distance.
Mathematically, work (W) is expressed as:
W = F ⋅ d ⋅ cos(θ)
where:
- F is the magnitude of the force,
- d is the magnitude of the displacement, and
- θ is the angle between the force and displacement vectors.
The Joule, therefore, directly measures the energy transferred by this interaction. One Joule of work is done when a force of one Newton displaces an object by one meter in the direction of the force.
Heat: Energy Transfer by Temperature Difference
Heat is another form of energy transfer, specifically driven by temperature differences. It is the flow of thermal energy from a hotter object to a cooler one. This transfer continues until thermal equilibrium is reached.
The Joule quantifies the amount of energy transferred as heat. For example, the specific heat capacity of a substance tells us how many Joules of energy are required to raise the temperature of one kilogram of that substance by one degree Celsius (or Kelvin).
Kinetic Energy: The Energy of Motion
Any object in motion possesses kinetic energy, which is directly proportional to its mass and the square of its velocity. This relationship is described by the equation:
KE = (1/2) m v2
where:
- KE represents kinetic energy,
- m represents mass, and
- v represents velocity.
The faster an object moves, and the more massive it is, the greater its kinetic energy—and the more Joules it embodies.
Potential Energy: Stored Energy Ready for Conversion
Potential energy represents stored energy that has the potential to be converted into other forms of energy, such as kinetic energy. It exists in various forms, including:
-
Gravitational Potential Energy: Energy stored due to an object's height above a reference point.
-
Elastic Potential Energy: Energy stored in a stretched or compressed spring.
-
Chemical Potential Energy: Energy stored in the bonds of molecules.
The Joule is used to quantify the amount of stored energy, reflecting the amount of work that could be done by releasing that energy. Understanding potential energy is crucial in analyzing systems where energy is conserved and transformed, powering much of the world around us.
Joules and Their Relatives: Navigating the Unit Landscape
Having established the fundamental role of the Joule in energy measurement, it's essential to contextualize its scale and relationships with other energy units. This involves understanding how SI prefixes are used to express smaller Joule quantities and comparing the Joule to other common units like calories, kilocalories, and electronvolts. This provides a more complete understanding of the "energy landscape."
Understanding Fractional Joules with SI Prefixes
The Joule, while fundamental, is often too large a unit for describing energies at the micro or nanoscale. To address this, the SI system employs prefixes to denote fractional quantities of the Joule.
MilliJoules (mJ), equivalent to one-thousandth of a Joule (10-3 J), are commonly encountered in the context of small electronic devices and sensors.
MicroJoules (µJ), representing one-millionth of a Joule (10-6 J), become relevant when analyzing the energy consumption of microcontrollers or MEMS devices.
At even smaller scales, nanoJoules (nJ) and picoJoules (pJ) are used to describe the energies associated with individual photons or quantum phenomena.
The use of these prefixes allows for a more convenient and intuitive representation of energy at different scales, avoiding the need for cumbersome scientific notation.
Joules Compared to Other Energy Units
While the Joule is the SI standard, other energy units persist in various fields due to historical reasons or practical considerations. Understanding how these units relate to the Joule is crucial for interpreting data and converting between different measurement systems.
Calories and Kilocalories
The calorie (cal), defined as the amount of energy required to raise the temperature of 1 gram of water by 1 degree Celsius, is still commonly used in the context of nutrition. One calorie is equivalent to approximately 4.184 Joules.
The kilocalorie (kcal), also known as the "large calorie" or "food calorie," is equal to 1000 calories and is used to express the energy content of food. Therefore, 1 kcal = 4184 J.
Although Joules are more scientifically accurate and consistently used in physics and engineering, the familiar calorie and kilocalorie remain entrenched in dietary contexts.
Electronvolts
The electronvolt (eV) is a unit of energy commonly used in atomic and nuclear physics. It represents the amount of energy gained by a single electron when it moves through an electric potential difference of one volt.
One electronvolt is equivalent to approximately 1.602 × 10-19 Joules. The electronvolt is particularly useful for expressing the energies of individual particles and photons, simplifying calculations in these specialized areas.
Conversion Factors: Bridging the Gap
To effectively work with different energy units, it's essential to be able to convert between them. Here are some key conversion factors:
- 1 cal = 4.184 J
- 1 kcal = 4184 J
- 1 eV = 1.602 × 10-19 J
Understanding and applying these conversion factors allows for seamless translation between different measurement systems, ensuring consistency and accuracy in energy-related calculations. This is particularly important when working with data from diverse sources or disciplines.
Energy in Various Forms: Thermal, Photon, and Threshold
Having established the fundamental role of the Joule in energy measurement, it's essential to contextualize its application across diverse forms of energy. This section delves into thermal energy, photon energy, and threshold energy, clarifying how the Joule is utilized to quantify these different manifestations of energy in various scientific and engineering contexts.
Thermal Energy: The Dance of Molecules
Thermal energy, often misunderstood, is the energy associated with the motion of atoms and molecules within a substance. This motion can be translational (moving from one place to another), rotational (spinning), or vibrational (oscillating). The higher the temperature of a substance, the greater the average kinetic energy of its constituent particles, and thus, the greater its thermal energy.
Mathematically, thermal energy changes are often calculated using the formula:
Q = mcΔT,
where Q is the heat transferred (in Joules), m is the mass of the substance, c is its specific heat capacity (in J/kg·K), and ΔT is the change in temperature (in Kelvin or Celsius).
The specific heat capacity is a critical property, representing the amount of energy required to raise the temperature of one kilogram of a substance by one degree Celsius. Water, for example, has a high specific heat capacity, making it an effective coolant.
Consider heating a cup of water on a stove. The electrical energy supplied to the heating element is converted into thermal energy, increasing the kinetic energy of the water molecules and raising the water's temperature.
Photon Energy: The Quantum of Light
Photon energy, a cornerstone of quantum mechanics, refers to the energy carried by a single photon, the fundamental particle of light. This energy is directly proportional to the frequency of the electromagnetic radiation and inversely proportional to its wavelength.
The relationship is described by the equation:
E = hf = hc/λ,
where E is the energy of the photon (in Joules), h is Planck's constant (approximately 6.626 x 10^-34 J·s), f is the frequency of the light (in Hertz), c is the speed of light (approximately 3.00 x 10^8 m/s), and λ is the wavelength of the light (in meters).
For example, a photon of blue light with a wavelength of 450 nm has a higher energy than a photon of red light with a wavelength of 700 nm.
This difference in photon energy is what dictates the color we perceive. Furthermore, understanding photon energy is critical in applications such as solar energy, where photons from the sun are harnessed to generate electricity.
Threshold Energy: The Key to Reactions
Threshold energy, also known as activation energy, is the minimum energy required for a chemical reaction to occur. Before reactants can transform into products, they must overcome an energy barrier. This barrier represents the energy needed to break existing bonds and initiate the formation of new ones.
Imagine pushing a rock over a hill. The threshold energy is analogous to the energy needed to push the rock to the top of the hill, after which it will roll down on its own.
Reactions with high threshold energies tend to be slow, while those with low threshold energies proceed more rapidly. Catalysts can lower the threshold energy of a reaction, thereby increasing the reaction rate.
In many industrial processes, optimizing reaction conditions to minimize energy input while ensuring that the threshold energy is met is critical for efficiency and sustainability.
Examples and Scales
To provide a sense of scale, consider these examples:
- Thermal Energy: The thermal energy required to raise the temperature of 1 gram of water by 1°C is approximately 4.18 Joules.
- Photon Energy: A photon of green light (550 nm) carries approximately 3.6 x 10^-19 Joules of energy.
- Threshold Energy: The activation energy for a typical chemical reaction might range from 50 to 200 kJ/mol (kilojoules per mole), which translates to around 8.3 x 10^-20 to 3.3 x 10^-19 Joules per molecule.
Understanding these different forms of energy and their corresponding scales, all measured in Joules, provides a comprehensive view of how energy manifests in various physical and chemical processes. The Joule, therefore, remains a powerful and versatile unit for quantifying energy across the scientific spectrum.
Micro-Joule Marvels: Devices Powered by Tiny Energies
Having explored the diverse forms of energy and the Joule’s role in quantifying them, we now turn our attention to devices that operate on minuscule energy budgets. This section illuminates a range of technologies, from microcontrollers to LEDs, that function effectively with fractions of a Joule. Understanding their operation reveals the practical significance of working with low-energy systems.
Microcontrollers: The Brains of Energy Efficiency
Microcontrollers, such as the ubiquitous Arduino and the increasingly popular Raspberry Pi Pico, exemplify energy-conscious design. These miniature computers serve as the brains behind countless embedded systems, from controlling household appliances to monitoring environmental conditions.
The energy consumption of a microcontroller is a critical factor in determining the overall efficiency of the device it controls. For instance, an Arduino Uno might consume around 50 millijoules per second when actively processing data.
Conversely, a low-power microcontroller like the Raspberry Pi Pico can operate in the single-digit millijoule range, especially when utilizing its sleep modes. This disparity highlights the importance of selecting the right microcontroller for the specific application and its energy constraints.
Sensors: Sensing the World with Minimal Power
Sensors are the eyes and ears of modern technology, continuously gathering data about their surroundings. From temperature and humidity to pressure and light, sensors provide crucial inputs for a wide array of systems.
The effectiveness of a sensor often hinges on its ability to operate with minimal energy consumption. A typical temperature sensor, for example, may only require a few microjoules per reading.
This low-energy operation is particularly critical for applications like wireless sensor networks, where sensors are deployed in remote locations and powered by batteries or energy harvesting devices. Reducing sensor power consumption extends battery life and minimizes maintenance.
RFID Tags: Powering Contactless Communication
Radio-Frequency Identification (RFID) tags are small, passive devices that enable contactless identification and tracking. Used extensively in retail, logistics, and security, RFID tags rely on energy-efficient data transmission to communicate with readers.
RFID tags operate by harvesting energy from the radio waves emitted by the reader. This harvested energy powers the tag's circuitry, allowing it to transmit its unique identification code.
The energy required for this transmission is remarkably low, typically in the nanojoule to microjoule range. Minimizing the energy needed for data transmission is essential for maximizing the read range and reliability of RFID systems.
Piezoelectric Generators/Sensors: Harvesting Mechanical Energy
Piezoelectric materials convert mechanical stress into electrical energy, and vice versa. This property is exploited in both piezoelectric generators and sensors. Piezoelectric generators harvest energy from vibrations or pressure, while piezoelectric sensors detect mechanical forces by generating an electrical signal.
The energy conversion efficiency of piezoelectric devices is crucial for their performance. A piezoelectric generator might produce microjoules of energy per vibration cycle, depending on the material properties and the magnitude of the applied force.
Similarly, a piezoelectric sensor can detect incredibly small forces, generating an electrical signal proportional to the applied stress.
MEMS Devices: Microscopic Machines with Minimal Energy Demands
Micro-Electro-Mechanical Systems (MEMS) are miniaturized mechanical and electromechanical devices that are fabricated using microfabrication techniques. These devices are used in a wide range of applications, including accelerometers, gyroscopes, and pressure sensors.
The operational scales in micro-energy environments are critical to MEMS device functionality. These devices operate with extremely low power consumption, often in the nanojoule to microjoule range.
This low power consumption enables MEMS devices to be integrated into battery-powered or energy-harvesting systems. For example, a MEMS accelerometer in a smartphone might consume only a few microjoules per measurement.
Laser Pointers: Precise Light with Fractional Joules
Laser pointers are seemingly simple devices that emit a focused beam of light. While they may appear power-hungry, modern laser pointers are surprisingly energy-efficient.
The energy per pulse usage in a laser pointer is typically in the millijoule range. This energy is used to excite the lasing medium, which then emits a coherent beam of light.
The efficiency of the laser diode and the optical components determines the overall power consumption of the laser pointer. More efficient designs can reduce the energy per pulse, extending battery life.
Medical Devices: Energy Efficiency for Patient Care
Medical devices are increasingly reliant on low-power electronics to improve patient care and reduce costs. From wearable sensors to implantable devices, energy efficiency is a critical consideration.
Many medical devices operate in the millijoule range, particularly those that are battery-powered or implanted. For example, a glucose monitor might consume a few millijoules per reading, while a cardiac pacemaker might require only a few microjoules per pulse.
Minimizing the energy consumption of medical devices is crucial for extending battery life, reducing the need for frequent replacements, and improving patient comfort.
Actuators and Solenoids: Converting Energy to Motion
Actuators and solenoids convert electrical energy into mechanical motion. Actuators are used to control the position or movement of a device, while solenoids are used to activate a mechanical switch or valve.
The energy conversion efficiency of actuators and solenoids is a critical factor in their performance. A small solenoid might require millijoules of energy to actuate, while a larger actuator might consume several joules.
The design of the actuator or solenoid determines its energy efficiency. More efficient designs can reduce the energy required for actuation, extending battery life and reducing operating costs.
LEDs: Illuminating the World with Minimal Energy
Light-Emitting Diodes (LEDs) have revolutionized the lighting industry due to their high efficiency and long lifespan. LEDs convert electrical energy directly into light, with minimal energy wasted as heat.
The minimal energy required for light emission in LEDs is a key factor in their energy efficiency. A typical LED might require only a few millijoules per second to produce a bright, visible light.
The efficiency of the LED depends on the semiconductor material and the manufacturing process. More efficient LEDs can produce more light with less energy, reducing energy consumption and extending lifespan.
Energy Consumption Values: A Comparative Overview
Device | Typical Energy Consumption |
---|---|
Arduino Uno | ~50 millijoules per second (active) |
Raspberry Pi Pico | Single-digit millijoules (sleep mode) |
Temperature Sensor | Few microjoules per reading |
RFID Tag | Nanojoule to microjoule range per transmission |
Piezoelectric Generator | Microjoules per vibration cycle |
MEMS Accelerometer | Few microjoules per measurement |
Laser Pointer | Millijoules per pulse |
Glucose Monitor | Few millijoules per reading |
Cardiac Pacemaker | Few microjoules per pulse |
Small Solenoid | Millijoules per actuation |
LED | Few millijoules per second |
This table provides a comparative overview of the typical energy consumption values for the devices discussed in this section. It highlights the diversity of energy requirements across different applications and underscores the importance of understanding and managing energy consumption in various electronic systems.
Practical Implications: Designing for Efficiency
Having explored the diverse forms of energy and the Joule’s role in quantifying them, we now turn our attention to devices that operate on minuscule energy budgets. This section illuminates a range of technologies, from microcontrollers to LEDs, that function effectively with fractions of a Joule. But beyond showcasing these marvels, we delve into the practical implications of understanding the Joule, particularly in the context of designing for efficiency.
The ability to precisely measure and manage energy at the micro and millijoule level is no longer a niche pursuit; it is a fundamental requirement for modern device engineering. It’s the cornerstone of creating sustainable, long-lasting, and high-performance electronic systems.
Low-Power Design: A Joule-Conscious Approach
Understanding the Joule allows engineers to make informed decisions at every stage of the design process, from component selection to software optimization. Every choice has an energy consequence, and a deep appreciation for the Joule helps quantify that consequence and guide trade-offs.
The key to designing for low-power consumption is a meticulous, data-driven approach. It requires thoroughly analyzing energy consumption at each level of the system. This includes not only the active components like processors and sensors but also the passive components and even the PCB traces.
Knowing how much energy each process consumes enables engineers to pinpoint inefficiencies. Once the sources of energy waste are identified, targeted optimization strategies can be implemented. These strategies range from choosing energy-efficient algorithms to employing power gating techniques that shut down unused parts of the circuit.
Energy Efficiency: The Cornerstone of Modern Engineering
Energy efficiency is more than just a desirable feature; it's a critical design constraint in today's world. Regulatory pressures, environmental concerns, and consumer demand for longer battery life are all converging to make energy efficiency a paramount concern.
Consider the proliferation of battery-powered devices, from smartphones and laptops to wearables and IoT sensors. In each case, energy efficiency directly translates to longer battery life and reduced environmental impact.
Moreover, energy-efficient designs often lead to smaller, lighter, and cooler devices. This is a significant advantage in applications where size and weight are critical, such as medical implants and portable electronics.
The push for energy efficiency also extends to the software realm. Efficient algorithms, optimized data structures, and intelligent power management techniques can dramatically reduce the energy consumption of software applications. In essence, software engineers are becoming energy managers.
Impact on Daily Life: A World Powered by Less
The benefits of low-power design and energy efficiency extend far beyond the realm of engineering and directly impact our daily lives. The ripple effects of these advancements are felt across various sectors, transforming how we interact with technology and our environment.
Extended Battery Life
Perhaps the most obvious benefit is longer battery life for our portable devices. This translates to fewer charging cycles, increased convenience, and reduced reliance on wall outlets. Imagine a world where your smartphone lasts for days on a single charge or your wearable device runs for weeks without needing a recharge.
Reduced Energy Consumption
Energy-efficient devices consume less power, lowering our electricity bills and reducing our carbon footprint. This is particularly important in developing countries, where access to reliable and affordable energy is often limited.
Sustainable Technology
By minimizing energy waste, we can create more sustainable technologies that are less harmful to the environment. This includes reducing the demand for scarce resources, minimizing electronic waste, and mitigating the effects of climate change.
New Possibilities
Finally, low-power design enables entirely new possibilities. Ultra-low-power sensors can be deployed in remote locations to monitor environmental conditions, track wildlife, or detect structural damage. Wireless sensor networks can be used to optimize energy consumption in buildings, improve agricultural yields, or enhance public safety. The possibilities are virtually endless.
In conclusion, the understanding and application of the Joule at the micro and millijoule level is not merely an academic exercise but a driving force behind technological innovation and sustainable development. It empowers engineers to create more efficient, more reliable, and more environmentally friendly devices that enrich our lives and protect our planet.
Video: Fraction of a Joule: Examples and Uses Explained
FAQs: Fraction of a Joule
What are some everyday examples of energy measurements involving a fraction of a joule?
Clicking a computer mouse or briefly lighting an LED are examples. These actions require very small amounts of energy, often measured as a fraction of a joule because a full joule would be excessive for such quick, low-power events.
How does understanding fractions of a joule help in electronics?
Electronics often deal with very small energy flows. Designing efficient circuits and devices requires precise understanding of energy consumption, down to a fraction of a joule. This allows engineers to optimize battery life and minimize energy waste.
Why are fractions of a joule important in scientific experiments?
Many scientific instruments measure tiny energy changes. High-precision calorimeters, for example, can detect heat changes in reactions that are only a fraction of a joule. This sensitivity is essential for accurate data collection in various fields.
What's the difference between a joule and a fraction of a joule?
A joule is a standard unit of energy. A fraction of a joule simply represents a smaller amount of energy, less than one joule. It's like comparing a dollar to a cent; a cent is a fraction of a dollar. The choice of unit depends on the scale of energy being measured.
So, the next time you're thinking about energy, remember it's not all about the big, flashy stuff like lightning strikes. Even tiny amounts of energy, like a fraction of a joule, play a crucial role in our everyday lives, powering everything from the sensors in your phone to the tiny sparks in a lighter. Pretty cool, right?