Glucose Fischer Projection: The Ultimate Visual Guide!
Understanding carbohydrate stereochemistry requires familiarity with the glucose Fischer projection, a critical tool for visualizing sugar molecules. The Fischer projection itself, attributed to chemist Emil Fischer, provides a 2D representation of a 3D molecule. This article clarifies how to interpret the glucose Fischer projection, connecting its structure to the properties relevant in areas like biochemistry, particularly within metabolic processes.
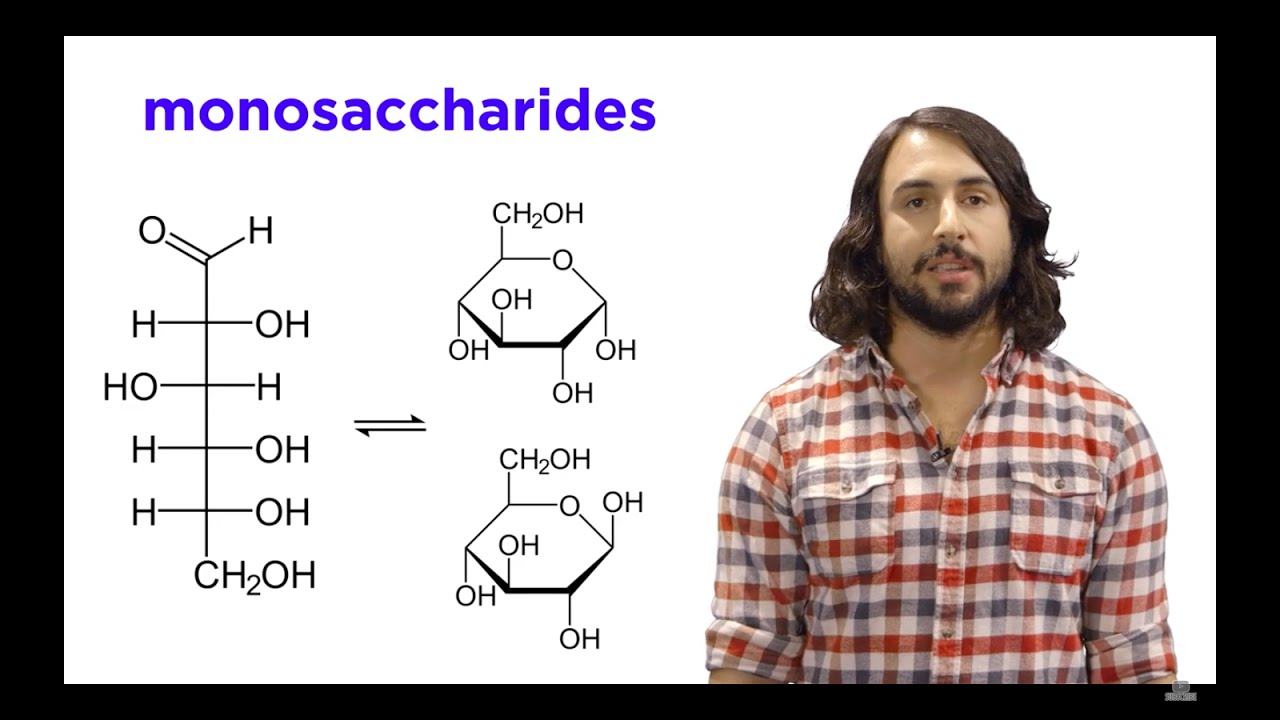
Image taken from the YouTube channel Professor Dave Explains , from the video titled Carbohydrates Part 1: Simple Sugars and Fischer Projections .
Glucose, a simple sugar also known as dextrose, stands as a cornerstone molecule in the realms of biology and chemistry. Its fundamental role as a primary energy source for living organisms and its involvement in a myriad of metabolic pathways underscore its significance. To truly understand the complex biochemical processes in which glucose participates, a firm grasp of its structure is essential.
Glucose: A Vital Monosaccharide
Glucose belongs to the class of carbohydrates known as monosaccharides, the simplest form of sugar.
It serves as the primary fuel for cellular respiration in most organisms, providing the energy necessary for life's processes. From the simplest bacteria to the most complex mammals, glucose plays an indispensable role in sustaining life.
The Importance of Understanding Glucose's Structure
Understanding the intricacies of glucose's structure is paramount for several reasons.
Firstly, its stereochemistry, or the three-dimensional arrangement of its atoms, dictates its specific biological activity. Subtle changes in this arrangement can drastically alter its function. Secondly, grasping glucose's structure allows us to comprehend how it interacts with other molecules, such as enzymes and receptors, within biological systems.
These interactions are critical for processes like glucose metabolism and signaling. Finally, by understanding the structure of glucose, we can better understand the structures of more complex carbohydrates.
The Fischer Projection: A Key Visualization Tool
The Fischer projection is a powerful tool used to represent the three-dimensional structure of glucose (and other chiral molecules) in a two-dimensional format.
Developed by Hermann Emil Fischer, this projection provides a simplified and standardized way to depict the stereochemistry of carbohydrates, especially the arrangement of hydroxyl groups around chiral carbon atoms. While it is a simplification, the Fischer projection allows chemists and biologists to easily visualize and compare the configurations of different stereoisomers of glucose.
Mastering the Fischer projection is crucial for effectively communicating and understanding the structure and reactivity of glucose in various chemical and biological contexts.
Glucose's structure, while complex, can be elegantly represented using the Fischer projection. It allows us to distill the three-dimensional arrangement of atoms into a readily understandable two-dimensional format. The Fischer projection is not merely a diagram; it is a key that unlocks deeper insights into glucose's behavior and interactions.
Decoding the Fischer Projection: A 2D Representation of a 3D Molecule
The Fischer projection serves as a standardized method for depicting three-dimensional organic molecules, particularly those with chiral centers, on a two-dimensional plane.
Its primary purpose is to simplify the representation of stereochemistry, making it easier to compare and analyze different stereoisomers. This projection is especially valuable in carbohydrate chemistry, where multiple chiral centers are common.
Unveiling the Purpose of the Fischer Projection
The Fischer projection transforms a tetrahedral carbon atom into a cross. Each chiral carbon in a molecule is represented by the intersection of two perpendicular lines. These intersections depict the central carbon atom.
The lines themselves represent bonds to substituent groups. This 2D rendering is a codified language chemists use to communicate the absolute configuration around a chiral center in a concise manner.
Deciphering the Conventions: Directionality in the Fischer Projection
The true power of the Fischer projection lies in its inherent conventions, which dictate the spatial orientation of the bonds. Vertical lines in a Fischer projection represent bonds that project away from the viewer, receding into the plane of the paper.
Conversely, horizontal lines represent bonds that project towards the viewer, coming out of the plane of the paper. This convention is crucial for accurately interpreting the stereochemistry depicted by the projection.
Understanding these conventions allows one to visualize the three-dimensional arrangement of atoms around each chiral center, which is vital for predicting a molecule's properties and reactivity. It's the key to converting the 2D representation back into a 3D mental image.
Remembering the Rules
A helpful mnemonic to recall these conventions is:
Vertical lines vanish away, horizontal lines hug you towards.
These conventions must be scrupulously followed to ensure an accurate representation of the molecule's stereochemistry. Flipping the projection by 90 degrees in the plane of the page inverts the stereochemistry at each chiral center and creates the enantiomer of the starting molecule.
The Legacy of Emil Fischer: A Foundation for Organic Chemistry
The Fischer projection owes its existence to the pioneering work of Hermann Emil Fischer, a Nobel laureate renowned for his contributions to organic chemistry. Fischer developed this projection method in the late 19th century as part of his groundbreaking research on sugars and enzymes.
His work revolutionized the field, providing chemists with a standardized way to represent and understand the stereochemistry of complex molecules. Fischer's projection method remains an indispensable tool in organic chemistry to this day.
It's a testament to the enduring impact of his work on our understanding of molecular structure and its relationship to biological activity. The Fischer projection is more than just a drawing; it's a piece of scientific history.
Decoding the Fischer projection allows us to translate the abstract symbols into a tangible understanding of glucose's structure. With this foundation in place, we can now explore the specific details that define glucose, setting the stage for understanding its unique properties and biological roles.
Glucose: An Aldohexose in Detail
Glucose isn't just any sugar; it's a meticulously structured molecule. At its heart, glucose is classified as an aldohexose. This designation reveals two key aspects of its structure: it's an aldose, meaning it contains an aldehyde group, and it's a hexose, indicating that it comprises six carbon atoms.
The Six-Carbon Arrangement
The six carbon atoms in glucose aren't simply strung together in a linear fashion. They form a backbone, a chain where each carbon atom is linked to its neighbors. This arrangement provides the fundamental framework upon which the rest of the molecule is built.
Carbon 1 is part of the aldehyde group (C=O), making it the most oxidized carbon in the molecule. Carbons 2 through 5 each bear a hydroxyl group (-OH), and the stereochemical orientation of these hydroxyl groups is what truly defines glucose. Carbon 6 is linked to carbon 5 and has two hydrogen atoms and one hydroxyl group.
Stereochemistry: The Key to Glucose's Identity
While the basic aldohexose structure provides a starting point, it's stereochemistry that distinguishes glucose from other similar sugars like galactose or mannose. Stereochemistry refers to the three-dimensional arrangement of atoms in a molecule.
Because glucose contains multiple chiral centers (specifically, carbons 2, 3, 4, and 5), it exists in various stereoisomeric forms. The specific configuration around these chiral carbons is critical to its biological activity. For instance, D-glucose, the naturally occurring form, is readily metabolized by living organisms, whereas its enantiomer, L-glucose, is not.
The precise spatial arrangement of the hydroxyl groups dictates how glucose interacts with enzymes and other biological molecules. This interaction is essential for its role as a primary energy source and a key building block in larger carbohydrates. The arrangement governs its properties and role in biological processes.
Decoding the Fischer projection allows us to translate the abstract symbols into a tangible understanding of glucose's structure. With this foundation in place, we can now explore the specific details that define glucose, setting the stage for understanding its unique properties and biological roles.
Drawing D-Glucose: A Step-by-Step Guide to Fischer Projections
The Fischer projection, while a simplified representation, is a powerful tool for visualizing and understanding the stereochemistry of sugars like D-glucose. Accurately drawing the Fischer projection of D-glucose is essential for communicating its structure clearly and unambiguously.
Here's a step-by-step guide to help you master this technique.
Step 1: Establishing the Carbon Backbone
Begin by drawing a vertical line. This line represents the carbon backbone of the glucose molecule. Since glucose is a hexose, meaning it has six carbon atoms, ensure your vertical line includes five intersections or crossing points. These intersections will represent carbons 2 through 6, while the top of the line and the bottom will designate carbons 1 and 6, respectively.
It's crucial that the vertical line is perfectly straight to avoid any misinterpretation of the structure. This backbone establishes the foundation for the rest of the drawing.
Step 2: Positioning the Carbonyl Group
The carbonyl group (C=O), in the form of an aldehyde, always occupies the top position in the Fischer projection of an aldose. This convention is crucial.
Draw the aldehyde group (CHO) at the top of the vertical line, indicating carbon 1. This placement signifies the starting point for numbering the carbon chain.
Step 3: Identifying and Representing Chiral Centers
Glucose has four chiral carbons (carbons 2, 3, 4, and 5). These are the carbons to which four different groups are attached, making them stereocenters.
Represent each chiral carbon with a horizontal line intersecting the vertical carbon backbone. These horizontal lines represent the bonds extending out of the plane of the page towards the viewer.
Step 4: Orienting the Hydroxyl Groups
This is where the specificity of D-glucose comes into play. The arrangement of the hydroxyl (-OH) groups on the chiral carbons defines D-glucose.
- Carbon 2: The -OH group is on the right.
- Carbon 3: The -OH group is on the left.
- Carbon 4: The -OH group is on the right.
- Carbon 5: The -OH group is on the right.
Place the -OH groups and hydrogen atoms (-H) accordingly on the horizontal lines extending from each chiral carbon. Remember, the horizontal lines indicate that these groups are pointing towards you.
Step 5: Completing Carbon 6
Finally, at the bottom of the vertical line, represent carbon 6 as a CH2OH group.
This completes the Fischer projection of D-glucose. The arrangement of the hydroxyl groups is paramount for accurately representing D-glucose.
Ensuring Accuracy: Key Considerations
Double-check that you've followed these key rules to avoid common mistakes:
- Vertical Line: Represents the carbon chain, with the most oxidized carbon (carbonyl group) at the top.
- Horizontal Lines: Represent bonds pointing towards the viewer.
- Hydroxyl Group Placement: Absolutely crucial for distinguishing D-glucose from other sugars.
- D-Configuration: Confirmed by the -OH group on the penultimate carbon (carbon 5) being on the right.
By carefully following these steps, you can confidently and accurately draw the Fischer projection of D-glucose, laying the foundation for understanding its chemical behavior and biological function. Mastering this skill is essential for delving deeper into carbohydrate chemistry and biochemistry.
Decoding the Fischer projection allows us to translate the abstract symbols into a tangible understanding of glucose's structure. With this foundation in place, we can now explore the specific details that define glucose, setting the stage for understanding its unique properties and biological roles.
D-Glucose vs. L-Glucose: Understanding Relative Configuration
While D-glucose is the naturally occurring and biologically relevant form, it's important to understand its relationship to its mirror image, L-glucose.
These two molecules are enantiomers, non-superimposable mirror images, much like your left and right hands. This difference, though subtle, has profound implications for their interactions with biological systems.
Enantiomers: Mirror Images in Molecular Form
Enantiomers are stereoisomers that are mirror images of each other. They possess identical physical properties, such as melting point and boiling point, but differ in how they interact with plane-polarized light and chiral environments, like enzymes.
D-glucose and L-glucose perfectly exemplify this relationship. Every chiral center in D-glucose has the opposite configuration in L-glucose.
This seemingly small difference dictates how these molecules are "recognized" by enzymes and other biological receptors.
Relative Configuration: D and L Nomenclature
The D and L nomenclature is a system of relative configuration used to designate the stereochemistry of carbohydrates and amino acids. It refers to the configuration of the chiral carbon farthest from the carbonyl group, which, in the case of glucose, is carbon number 5.
-
D-Glucose: If the hydroxyl group (-OH) on this penultimate carbon (C5) points to the right in the Fischer projection, the sugar is designated as D.
-
L-Glucose: Conversely, if the -OH group on C5 points to the left, the sugar is designated as L.
It's important to note that the D and L designations do not indicate the direction in which the molecule rotates plane-polarized light (dextrorotatory (+) or levorotatory (-)). The D/L designation is based solely on the configuration at the penultimate carbon.
Biological Significance: A Tale of Two Isomers
The biological world exhibits a strong preference for D-glucose. Enzymes involved in glycolysis and other metabolic pathways are highly specific for D-glucose, efficiently processing it for energy.
L-glucose, on the other hand, is not readily metabolized by most organisms. It is not a naturally occurring sugar in most biological systems.
Consequently, L-glucose has been explored as a low-calorie sweetener. Since it's not easily metabolized, it provides sweetness without contributing significantly to energy intake.
The differing biological activities of D-glucose and L-glucose highlight the importance of stereochemistry in determining the fate and function of molecules within living systems.
Decoding the dance between D- and L-glucose unveils the significance of molecular handedness in biological systems. But the story of glucose’s unique properties doesn’t end with relative configuration. It extends into the broader realm of chirality, stereoisomerism, and the fascinating phenomenon of optical activity, revealing the intricate details that govern its interactions with the world.
Chirality, Stereoisomers, and Optical Activity: Delving Deeper into Glucose's Properties
Chirality: The Handedness of Molecules
Chirality, derived from the Greek word for hand, describes a property of molecules that cannot be superimposed on their mirror images. Just as your left and right hands are mirror images but not identical, chiral molecules exist in two forms that are mirror images of each other.
The cornerstone of chirality in organic molecules is the presence of a chiral center, typically a carbon atom bonded to four different substituents.
Glucose, with its multiple asymmetric carbons, is a prime example of a chiral molecule, meaning it exists as non-superimposable mirror images.
Asymmetric Carbons and Stereoisomers
The asymmetric carbons within glucose are the genesis of its diverse stereoisomeric forms. Each chiral center can have two different spatial arrangements of its substituents, leading to a multitude of possible stereoisomers.
Stereoisomers are molecules that have the same molecular formula and sequence of bonded atoms, but differ in the three-dimensional orientations of their atoms in space.
The number of possible stereoisomers for a molecule is 2n, where n is the number of chiral centers. Glucose, possessing four chiral carbons, has 24 = 16 stereoisomers.
These stereoisomers can be further classified into enantiomers, diastereomers, and epimers, each with distinct relationships and properties.
Enantiomers, Diastereomers, and Epimers: A Stereoisomeric Family
Enantiomers
As previously discussed, enantiomers are stereoisomers that are non-superimposable mirror images of each other. D-glucose and L-glucose are the classic example.
They share identical physical properties, except for their interaction with polarized light, which will be elaborated on later.
Diastereomers
Diastereomers are stereoisomers that are not mirror images of each other. They differ in configuration at one or more, but not all, chiral centers.
Unlike enantiomers, diastereomers have different physical properties, such as melting point, boiling point, and solubility.
Epimers
Epimers are a special type of diastereomer that differ in configuration at only one chiral center. For instance, D-glucose and D-galactose are epimers, differing only at carbon 4. This seemingly minor difference leads to significant biological consequences.
Optical Activity: The Interaction with Light
Chiral molecules possess the remarkable ability to rotate the plane of plane-polarized light, a phenomenon known as optical activity.
When plane-polarized light passes through a solution of a chiral compound, the plane of polarization is rotated either clockwise (dextrorotatory, denoted as + or d) or counterclockwise (levorotatory, denoted as – or l).
Enantiomers rotate plane-polarized light to the same extent but in opposite directions. A racemic mixture, containing equal amounts of both enantiomers, shows no net rotation because the effects cancel each other out. The specific rotation is an important physical constant that provides information about the optical purity of a chiral compound.
Glucose, being a chiral molecule, is optically active. This property is crucial in identifying and characterizing glucose in various solutions and biological samples.
Decoding the dance between D- and L-glucose unveils the significance of molecular handedness in biological systems. But the story of glucose’s unique properties doesn’t end with relative configuration. It extends into the broader realm of chirality, stereoisomerism, and the fascinating phenomenon of optical activity, revealing the intricate details that govern its interactions with the world.
Beyond the Fischer Projection: Visualizing Glucose in 3D
The Fischer projection serves as an invaluable tool for representing stereochemistry, but it's crucial to acknowledge its inherent limitations. As a two-dimensional representation of a fundamentally three-dimensional molecule, it can sometimes obscure the true spatial relationships and conformational preferences of glucose. This is where alternative visualization methods come into play.
The Limitations of 2D Representations
The Fischer projection excels at depicting relative configurations around chiral centers. However, it inherently flattens the molecule, distorting bond angles and failing to convey the dynamic, flexible nature of glucose in solution.
It implies that all bonds are eclipsed, which is rarely the case in reality due to steric hindrance. Understanding these constraints is vital for avoiding misinterpretations of glucose's behavior and reactivity.
Molecular Models: A Tangible Approach
Molecular models offer a more intuitive and realistic way to visualize glucose's three-dimensional structure. These models, whether physical or digital, allow for the manipulation and rotation of the molecule, providing a direct sense of its spatial arrangement.
Ball-and-stick models clearly depict the connectivity and bond angles, while space-filling models illustrate the overall shape and volume occupied by the molecule. Using molecular models fosters a deeper understanding of steric interactions and accessibility.
Structural Formulas: Revealing Conformation
Structural formulas, including Haworth projections and conformational representations, provide a more nuanced depiction of glucose's three-dimensional arrangement.
Haworth projections, while still somewhat simplified, represent the cyclic form of glucose and highlight the axial or equatorial positions of substituents.
Conformational representations, such as chair conformations, offer the most accurate portrayal of glucose's preferred shape, showcasing the minimized steric strain.
Advantages of 3D Visualization
Visualizing glucose in three dimensions offers several key advantages. It allows for a more accurate understanding of its conformation, including ring puckering and the relative orientation of substituents.
This is critical for understanding glucose's interactions with enzymes and other biomolecules, as these interactions are highly dependent on the precise spatial arrangement of atoms.
Furthermore, 3D visualization helps to grasp the concept of conformational flexibility, acknowledging that glucose is not a static molecule but rather exists in a dynamic equilibrium of different conformations.
By moving beyond the Fischer projection and embracing these alternative visualization methods, we gain a more complete and nuanced understanding of glucose's structure and behavior. This, in turn, allows for a deeper appreciation of its role in biological systems.
Beyond unraveling the intricacies of its structure, it's crucial to place glucose within the broader context of carbohydrate chemistry. By understanding its position in the larger framework, we can appreciate its ubiquitous role in biological systems and its profound impact on life itself.
Glucose in the Grand Scheme: Carbohydrates and Monosaccharides
Glucose: A Cornerstone of Carbohydrate Chemistry
Carbohydrates, often called saccharides, represent a vast class of organic compounds essential for life. They serve as primary energy sources, structural components, and play crucial roles in cell signaling and recognition.
These vital compounds are broadly classified into monosaccharides, disaccharides, oligosaccharides, and polysaccharides, based on the number of sugar units they contain.
Glucose, a simple sugar, takes its place as a monosaccharide, the foundational building block of more complex carbohydrates.
From Simple to Complex: Building with Glucose
Monosaccharides, like glucose, are the simplest form of carbohydrates, unable to be further hydrolyzed into smaller sugar units.
They serve as the monomers from which disaccharides (two monosaccharides joined together, such as sucrose or lactose) and polysaccharides (long chains of many monosaccharides, such as starch, cellulose, and glycogen) are constructed.
In essence, glucose is a fundamental unit, much like a brick in a building, contributing to a wide array of complex carbohydrate structures with diverse functions.
Glucose: The Primary Energy Currency of Life
One of the most critical roles of glucose is its function as a primary energy source for living organisms.
Through the process of cellular respiration, glucose is broken down to release energy in the form of ATP (adenosine triphosphate), the universal energy currency of cells.
This energy fuels a myriad of cellular processes, from muscle contraction and nerve impulse transmission to protein synthesis and active transport.
The efficiency and accessibility of glucose as an energy source have made it a cornerstone of metabolic pathways across diverse life forms.
Glucose's Central Role in Metabolic Pathways
Glucose is not only a source of energy but also a key participant in numerous metabolic pathways.
Glycolysis, the initial stage of glucose metabolism, is a universal pathway that occurs in nearly all living cells. It breaks down glucose into pyruvate, generating a small amount of ATP and NADH (nicotinamide adenine dinucleotide).
Pyruvate can then be further metabolized through aerobic or anaerobic pathways, depending on the availability of oxygen.
Furthermore, glucose plays a central role in gluconeogenesis (the synthesis of glucose from non-carbohydrate precursors) and glycogenesis (the synthesis of glycogen for glucose storage).
These intricate metabolic pathways underscore glucose's importance in maintaining energy homeostasis and supporting cellular function.
By understanding how glucose fits into the broader categories of carbohydrates and its central role as an energy source, one can better appreciate its foundational position in biology and biochemistry.
Video: Glucose Fischer Projection: The Ultimate Visual Guide!
FAQs: Understanding Glucose Fischer Projections
Here are some frequently asked questions about glucose Fischer projections to help solidify your understanding.
What is a Fischer projection and why is it used for glucose?
A Fischer projection is a two-dimensional representation of a three-dimensional molecule, commonly used in organic chemistry. For glucose, it simplifies showing the stereochemistry of chiral centers, making it easier to compare different sugars. The glucose fischer projection provides a clear visual representation.
How do you determine the D or L configuration of glucose from its Fischer projection?
Look at the chiral carbon furthest from the aldehyde group (the bottom chiral carbon in the projection). If the -OH group is on the right, it's D-glucose. If it's on the left, it's L-glucose. This is a standard convention when drawing the glucose fischer projection.
What does a horizontal line and vertical line represent in the glucose fischer projection?
Horizontal lines in a Fischer projection represent bonds projecting out of the page, towards the viewer. Vertical lines represent bonds projecting behind the page, away from the viewer. This helps visualize the 3D arrangement of substituents in the glucose molecule depicted in the glucose fischer projection.
Can you easily convert a Fischer projection of glucose to its Haworth projection?
While the Fischer projection provides stereochemical information, converting it directly to a Haworth projection requires visualizing the ring closure and the orientation of the -OH groups on the Fischer projection. The positions on the right in the glucose fischer projection will point down in the Haworth projection if the carbon chain is numbered from the aldehyde group.
So there you have it! Hopefully, this guide helped demystify the glucose Fischer projection a little. Now you can confidently tackle those organic chemistry diagrams!