Tronegativity: Influence Bond Formation?
Tronegativity, a concept pioneered by Professor Reedijk at Leiden University, challenges conventional understanding of electronegativity by focusing on the ability of certain ligands to draw electron density away from a central metal atom. This phenomenon is particularly evident in coordination complexes analyzed through techniques like X-ray crystallography, where bond lengths and angles provide direct evidence of electron density distribution, impacting the stability and reactivity of the complex. Molecular orbital theory, specifically applied using computational tools such as Gaussian, helps to predict and rationalize how tronegativity influence bond formation?, affecting the electronic structure and properties of these compounds. The interplay between these factors ultimately dictates whether a bond will form and how strong it will be, offering insights into designing molecules with specific properties.
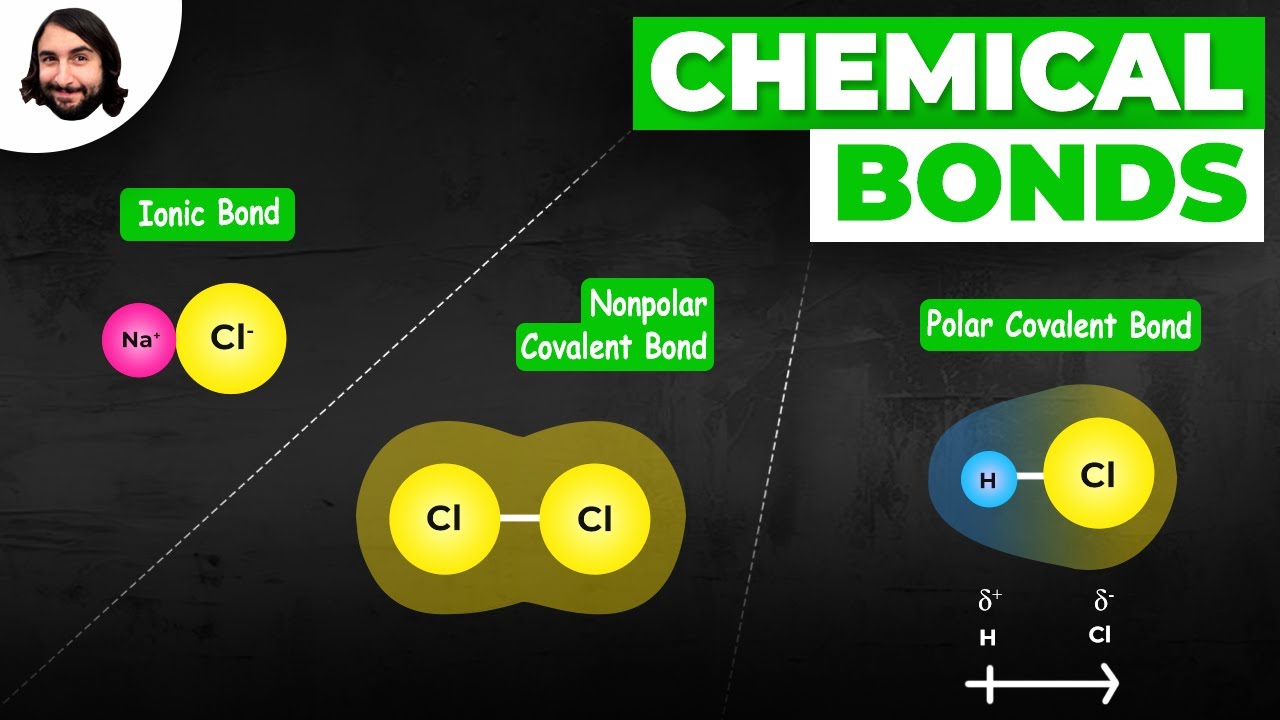
Image taken from the YouTube channel Professor Dave Explains , from the video titled The Chemical Bond: Covalent vs. Ionic and Polar vs. Nonpolar .
Decoding Tronegativity: A New Perspective on Chemical Bonding
The behavior of molecules, from their reactivity to their stability, is governed by the intricate dance of electrons. At the heart of this dance lies the concept of electronegativity, a fundamental property that quantifies an atom's ability to attract electrons within a chemical bond.
However, the traditional understanding of electronegativity, while valuable, often falls short of capturing the full complexity of electron distribution in real-world molecular environments.
Enter tronegativity, a refined and context-aware descriptor that offers a powerful new lens through which to examine chemical bonding.
Defining Tronegativity: Beyond Elemental Properties
Tronegativity, unlike its predecessor, is not merely an inherent property of an element. Rather, it is a measure of an atom's electron-attracting power within a specific molecular context. This context includes the atom's bonding partners, the overall molecular geometry, and the surrounding electronic environment.
In essence, tronegativity recognizes that an atom's ability to attract electrons is not static, but is dynamically influenced by its molecular surroundings.
The Significance of Tronegativity in Modern Chemistry
The advent of tronegativity marks a significant step forward in our ability to accurately model and predict molecular behavior. By considering the nuanced interplay of factors that influence electron distribution, tronegativity allows chemists to:
- Gain deeper insights into the nature of chemical bonds.
- More accurately predict reaction pathways.
- Design novel molecules with tailored properties.
This is particularly crucial in fields such as catalysis, drug design, and materials science, where precise control over molecular interactions is paramount.
Tronegativity vs. Electronegativity: A Nuanced Approach
While electronegativity provides a useful starting point, it often relies on simplified assumptions that can lead to inaccuracies. Traditional electronegativity scales, such as the Pauling and Mulliken scales, assign a fixed electronegativity value to each element, regardless of its molecular environment.
Tronegativity, on the other hand, accounts for the dynamic nature of electron distribution. It considers factors such as:
- Molecular geometry.
- Steric hindrance.
- The presence of electron-withdrawing or electron-donating groups.
By incorporating these contextual factors, tronegativity provides a more accurate and nuanced picture of electron distribution within a molecule. It allows chemists to move beyond simple generalizations and to understand the subtle forces that govern molecular behavior.
The Importance of Understanding Tronegativity
Understanding tronegativity is no longer a theoretical exercise; it is a practical necessity for researchers seeking to push the boundaries of chemical knowledge.
By embracing this refined concept, chemists can:
- Develop a more sophisticated understanding of molecular interactions.
- Design new catalysts with enhanced activity and selectivity.
- Create novel materials with tailored electronic and optical properties.
In short, tronegativity empowers chemists to predict and control molecular behavior with greater precision than ever before. This has profound implications for a wide range of applications, from developing life-saving drugs to creating sustainable energy solutions.
Tronegativity vs. Electronegativity: Unveiling the Differences
The behavior of molecules, from their reactivity to their stability, is governed by the intricate dance of electrons. At the heart of this dance lies the concept of electronegativity, a fundamental property that quantifies an atom's ability to attract electrons within a chemical bond. However, the classical understanding of electronegativity, while foundational, often falls short when applied to complex molecular environments. Tronegativity emerges as a sophisticated refinement, offering a more nuanced perspective on electron distribution and molecular behavior.
The Shortcomings of Traditional Electronegativity
Traditional electronegativity scales, such as those developed by Pauling and Mulliken, provide a valuable framework for understanding the relative electron-attracting abilities of different elements. These scales are based on atomic properties and offer a general guide for predicting bond polarity and reactivity.
However, these approaches are inherently limited. They treat electronegativity as an intrinsic atomic property, neglecting the influence of the surrounding molecular environment. In reality, an atom's electron-attracting ability is significantly affected by its bonding partners, molecular geometry, and the overall electronic structure of the molecule.
Traditional electronegativity values are essentially context-independent. This can lead to inaccurate predictions, particularly in molecules with complex bonding patterns, steric hindrance, or significant variations in electron density distribution.
Tronegativity: A Context-Aware Approach
Tronegativity addresses the limitations of traditional electronegativity by considering the specific molecular environment surrounding an atom. It acknowledges that an atom's electron-attracting ability is not a fixed property but rather a dynamic characteristic that varies depending on its chemical context.
This context-awareness is achieved by incorporating factors such as:
-
Molecular geometry: The spatial arrangement of atoms within a molecule influences electron density distribution and, consequently, the electron-attracting ability of individual atoms.
-
Steric hindrance: Bulky groups surrounding an atom can hinder its ability to interact with electrons, affecting its tronegativity.
-
Electron density distribution: The overall distribution of electrons within a molecule impacts the electronic environment around each atom, influencing its ability to attract additional electrons.
By incorporating these factors, tronegativity provides a more accurate representation of electron distribution and chemical behavior in complex molecules.
Illustrative Examples: Where Tronegativity Excels
Consider carbonyl compounds (aldehydes and ketones). Electronegativity alone struggles to fully explain the nuanced reactivity differences observed between various carbonyls. Tronegativity, however, accounts for the electronic effects of substituents attached to the carbonyl group, providing a more accurate prediction of reactivity towards nucleophilic attack.
Another compelling example can be found in organometallic chemistry. The bonding between a metal center and its ligands is highly dependent on the electronic environment created by the ligands. Traditional electronegativity values may fail to capture the subtle variations in metal-ligand bond polarity, while tronegativity, by considering the specific electronic properties of the ligands, offers a more accurate description of the bonding interactions.
Tronegativity: Refinement, Not Replacement
It is crucial to emphasize that tronegativity is not intended to replace traditional electronegativity. Instead, it should be viewed as a refinement and extension of the fundamental concept.
Electronegativity remains a valuable tool for providing a general understanding of chemical bonding and reactivity. Tronegativity builds upon this foundation by providing a more sophisticated and context-aware approach for analyzing electron distribution in complex molecular systems.
In essence, tronegativity empowers chemists with a more precise lens through which to view the intricate electronic landscape of molecules, leading to improved predictions and a deeper understanding of chemical behavior.
Foundational Concepts: Electron Density, Steric Hindrance, and Molecular Shape
[Tronegativity vs. Electronegativity: Unveiling the Differences The behavior of molecules, from their reactivity to their stability, is governed by the intricate dance of electrons. At the heart of this dance lies the concept of electronegativity, a fundamental property that quantifies an atom's ability to attract electrons within a chemical bond. H...] However, tronegativity moves beyond this simplified view by incorporating the complex interplay of electron density, steric hindrance, and molecular shape, offering a more nuanced understanding of chemical interactions.
Electron Density: The Engine of Molecular Properties
At the core of tronegativity lies the concept of electron density – the probability of finding an electron at a specific point in space. It's not merely about how many electrons an atom possesses, but how those electrons are distributed around the atom within the molecular framework.
This distribution dictates a wide range of crucial molecular properties. Bond strength, polarity, and reactivity are all directly influenced by the subtle variations in electron density.
A molecule with evenly distributed electron density might be stable and unreactive. Conversely, a molecule with regions of high and low electron density will likely exhibit polarity, making it susceptible to chemical attack and reactions.
Tronegativity's Gaze: How Electron Density Shapes Atomic Interactions
Tronegativity calculations explicitly consider the influence of electron density around an atom within a specific molecule. Unlike traditional electronegativity, which assigns a fixed value to each element, tronegativity acknowledges that an atom's electron-attracting power is not static. It is dynamically affected by its chemical environment.
Imagine a carbon atom bonded to three highly electronegative fluorine atoms versus a carbon atom bonded to three hydrogen atoms. In the first case, the carbon atom experiences a significant withdrawal of electron density. This alters its tronegativity value. It becomes more electropositive than it would be in the second scenario.
Steric Hindrance and Conformational Preferences: The Spatial Dance of Atoms
Steric hindrance arises when bulky groups surrounding an atom impede its ability to interact effectively with other atoms or molecules. This spatial constraint influences electron density distribution and, consequently, tronegativity values.
Consider a reaction site shielded by large alkyl groups. These groups can prevent an atom from approaching another, effectively lowering its tronegativity value in certain areas of the molecule.
Conformational preferences also play a crucial role. The preferred arrangement of atoms in a molecule can impact the local electron density distribution, altering the tronegativity of individual atoms.
Molecular Shape: A Blueprint for Chemical Behavior
Molecular shape, dictated by factors like bond angles and bond lengths, is intimately linked to chemical properties. It determines how a molecule interacts with its surroundings. It also influences its reactivity, solubility, and even its biological activity.
Tronegativity helps predict these properties by providing insights into the electron density distribution within the molecule's specific shape.
For example, a bent molecule may exhibit a different tronegativity profile compared to a linear molecule, even if they are composed of the same atoms. This difference in tronegativity can then explain variations in chemical behavior.
By understanding how electron density, steric hindrance, and molecular shape collectively influence atomic interactions, tronegativity provides a more holistic and accurate picture of chemical bonding. It moves beyond simple electronegativity and ultimately unlocks a deeper understanding of the molecular world.
Computational Methods: Calculating Tronegativity with DFT
The behavior of molecules, from their reactivity to their stability, is governed by the intricate dance of electrons. At the heart of this dance lies the concept of electronegativity, a fundamental property that, while useful, sometimes falls short in capturing the complexity of electron distribution in real-world molecular environments. This necessitates the use of sophisticated computational methods, particularly Density Functional Theory (DFT), to determine tronegativity values and gain a deeper understanding of molecular behavior.
Density Functional Theory: A Cornerstone of Tronegativity Calculations
DFT has emerged as a pivotal tool in computational chemistry, offering a powerful framework for modeling electronic structures and predicting molecular properties. Unlike traditional ab initio methods that solve the many-body Schrödinger equation directly, DFT focuses on the electron density, a more manageable quantity that nonetheless encapsulates all the information needed to determine the ground state energy and other properties of a system.
This approach drastically reduces the computational cost, making it feasible to study larger and more complex molecules.
At its core, DFT relies on the Hohenberg-Kohn theorems, which establish the unique relationship between the ground state electron density and the external potential acting on the system. In essence, if you know the electron density, you know everything about the molecule.
The DFT Workflow for Tronegativity Determination
Calculating tronegativity values using DFT involves a series of well-defined computational steps:
-
Molecular Geometry Optimization: The first step is to determine the most stable three-dimensional structure of the molecule. This is achieved by iteratively adjusting the positions of the atoms until the energy of the system is minimized.
-
Electronic Structure Calculation: With the optimized geometry in hand, a DFT calculation is performed to determine the electron density distribution. This involves solving the Kohn-Sham equations, a set of self-consistent equations that describe the behavior of electrons in the molecule.
-
Tronegativity Evaluation: Tronegativity is typically extracted from the calculated electron density or related properties, such as the electrostatic potential or the electron localization function. Different approaches exist for relating these quantities to tronegativity, each with its own strengths and limitations.
-
Analysis and Interpretation: The calculated tronegativity values are then analyzed and interpreted in the context of the molecular structure and properties. This may involve comparing tronegativity values for different atoms within the molecule, or comparing the tronegativity of the same atom in different molecular environments.
Popular Software Packages for DFT Calculations
The power of DFT is readily accessible through various computational chemistry software packages, each offering a range of functionalities and algorithms for electronic structure calculations:
-
Gaussian: A widely used commercial software package known for its versatility and comprehensive suite of methods.
-
ORCA: A popular choice for its user-friendliness and focus on spectroscopic properties and excited-state calculations.
-
NWChem: An open-source software package developed by the Pacific Northwest National Laboratory, offering a broad range of capabilities for high-performance computing.
-
VASP: Primarily used for solid-state physics calculations, VASP is also employed in computational chemistry, particularly for studying surface reactions and catalysis.
The Crucial Role of Computational Chemists and Molecular Modelers
The interpretation and application of tronegativity, grounded in DFT, are heavily reliant on the expertise of computational chemists and molecular modelers. These professionals play a crucial role in:
- Designing and executing DFT calculations: Selecting appropriate computational methods, basis sets, and other parameters to ensure accurate and reliable results.
- Analyzing and interpreting the results: Extracting meaningful insights from the vast amount of data generated by DFT calculations.
- Validating theoretical predictions with experimental data: Comparing calculated tronegativity values with experimental measurements to assess the accuracy of the calculations.
- Developing new and improved methods for calculating tronegativity: Pushing the boundaries of computational chemistry to provide increasingly accurate and reliable predictions of molecular behavior.
In essence, it is the collaborative effort of researchers and advanced computational tools that continues to illuminate the nuanced world of electron density and its profound influence on the chemical stage.
Applications of Tronegativity: Predicting Molecular Behavior
Computational Methods: Calculating Tronegativity with DFT The behavior of molecules, from their reactivity to their stability, is governed by the intricate dance of electrons. At the heart of this dance lies the concept of electronegativity, a fundamental property that, while useful, sometimes falls short in capturing the complexity of electron distributions within the nuanced environments of molecular structures. Here, we delve into how tronegativity emerges as a refined tool, enhancing our ability to foresee molecular conduct across various chemical scenarios.
Unveiling Predictive Power: Reactivity, Stability, and Beyond
Tronegativity, with its capacity to reflect the local electronic environment of an atom within a molecule, allows us to predict a spectrum of critical molecular behaviors. Foremost among these is chemical reactivity.
By identifying regions of high or low tronegativity, we can pinpoint sites prone to electrophilic or nucleophilic attack, paving the way for informed catalyst design and targeted synthesis strategies. Furthermore, tronegativity serves as a key indicator of molecular stability.
For instance, molecules featuring atoms with balanced tronegativity values often exhibit enhanced stability due to optimized charge distribution and reduced internal strain. Beyond reactivity and stability, tronegativity plays a pivotal role in forecasting other vital molecular traits, including acidity/basicity, bond strength, and even spectroscopic properties.
Case Studies: Tronegativity in Action
The true power of tronegativity resides in its successful application to explain and predict real-world chemical phenomena. Consider, for example, the regioselectivity observed in electrophilic aromatic substitution reactions.
Traditional electronegativity often falls short in rationalizing the observed product distribution, particularly when substituents with subtle electronic effects are involved. However, by employing tronegativity calculations, which account for the nuanced electronic environment around the aromatic ring, it becomes possible to accurately predict the preferred site of substitution.
Another compelling case involves the rationalization of bond strengths in transition metal complexes. Tronegativity can help explain variations in metal-ligand bond strength based on the specific electronic characteristics of the ligands and the metal center’s oxidation state and coordination geometry.
Such insight is invaluable in the design of novel catalysts and materials with tailored properties. It also can be applied in rationalizing the acidity of organic molecules. By understanding the tronegativity of atoms directly bonded to acidic protons, scientists can better predict and modify the acidity of compounds in organic synthesis.
Acknowledging Limitations: Tronegativity's Imperfect Lens
While tronegativity offers a significant leap forward in our ability to predict molecular behavior, it is crucial to recognize its limitations. It is not a panacea, and its predictive power is contingent on several factors. One critical aspect is the accuracy of the underlying computational methods used to calculate tronegativity values.
As discussed earlier, Density Functional Theory (DFT) is a primary tool in this regard, but DFT calculations are themselves subject to approximations and limitations. The choice of functional, basis set, and other computational parameters can influence the resulting tronegativity values, potentially leading to discrepancies between theoretical predictions and experimental observations.
Navigating Complex Systems: Challenges and Considerations
The application of tronegativity becomes increasingly challenging as we venture into the realm of large and complex molecular systems.
The computational cost associated with DFT calculations scales rapidly with system size, making it computationally demanding to accurately determine tronegativity values for macromolecules, proteins, or extended solid-state structures. In such cases, approximations and simplifications may be necessary, which can compromise the accuracy of the predictions.
Furthermore, in complex systems, other factors, such as solvation effects, dynamic conformational changes, and intermolecular interactions, can significantly influence molecular behavior.
These factors are not always fully captured by tronegativity calculations alone, necessitating the integration of other theoretical and experimental approaches. It is crucial to validate tronegativity-based predictions with experimental data whenever possible. Spectroscopic measurements, reactivity studies, and other experimental techniques can provide valuable insights into the electronic structure and behavior of molecules, helping to refine and improve our understanding of tronegativity.
Limitations and Challenges: A Critical Perspective on Tronegativity
The behavior of molecules, from their reactivity to their stability, is governed by the intricate dance of electrons. At the heart of this dance lies the concept of electronegativity, a fundamental property that, while useful, requires further refinement when considering the nuanced electronic environment within a molecule. Tronegativity emerges as a powerful tool to address these shortcomings. However, like any theoretical construct relying on computational methods, it is essential to acknowledge its inherent limitations and challenges.
Inherent Approximations in DFT Calculations
Tronegativity values, predominantly derived from Density Functional Theory (DFT) calculations, are not without their own set of approximations. DFT, while remarkably accurate for many systems, relies on approximations to the exchange-correlation functional.
These approximations, necessary to make the calculations computationally tractable, can introduce errors in the predicted electron density distribution, which directly impacts the calculated tronegativity values. The choice of functional, basis set, and other computational parameters can significantly influence the results.
Therefore, it's crucial to critically evaluate the computational setup and understand the potential sources of error when interpreting tronegativity values.
Discrepancies Between Calculated and Experimental Results
A persistent challenge in computational chemistry lies in the potential for discrepancies between theoretical predictions and experimental observations. While tronegativity offers a valuable perspective, its calculated values might not always perfectly align with experimentally derived measures of electron distribution or reactivity.
Several factors can contribute to these discrepancies. Experimental conditions (solvent effects, temperature) might not be fully accounted for in the calculations.
Furthermore, the inherent limitations of DFT, as discussed earlier, can lead to inaccuracies in the predicted electron density. It is important to remember that computational models are simplifications of reality, and a degree of deviation is almost inevitable.
Computational Cost for Large and Complex Molecules
The application of tronegativity concepts, particularly through DFT calculations, faces significant hurdles when dealing with large and complex molecules. The computational cost of DFT scales steeply with the number of atoms.
This can make accurate tronegativity calculations for large biomolecules or complex materials prohibitively expensive, even with modern high-performance computing resources.
Approximations and simplifications might be necessary to reduce the computational burden, but these can compromise the accuracy of the results. Developing more efficient algorithms and computational strategies is crucial for extending the applicability of tronegativity to more complex systems.
The Importance of Experimental Validation
Given the inherent limitations and potential sources of error, experimental validation is paramount for ensuring the reliability and accuracy of tronegativity predictions. Comparison with experimental data, such as spectroscopic measurements, X-ray diffraction analysis, or reactivity studies, is essential for validating the computational results.
Furthermore, comparison with other theoretical methods, such as ab initio calculations or quantum Monte Carlo simulations, can provide additional confidence in the accuracy of the tronegativity values.
A multifaceted approach, combining computational modeling with experimental validation, is key to realizing the full potential of tronegativity as a predictive tool in chemistry.
Video: Tronegativity: Influence Bond Formation?
FAQs: Tronegativity: Influence Bond Formation?
What is tronegativity and how does it differ from electronegativity?
Tronegativity is a recently proposed atomic property measuring an atom's ability to draw electron density towards itself in a chemical bond, considering the 3D shape of the molecule. While electronegativity focuses on inherent atomic properties, tronegativity influence bond formation by incorporating geometrical context. It offers a more detailed description of electron distribution in complex molecules.
How does tronegativity influence bond formation compared to electronegativity?
While electronegativity predicts bond polarity based on inherent atomic attraction, tronegativity influence bond formation by considering the 3D geometry surrounding the atom. For example, it can explain why a carbonyl group can be highly polarized even if carbon and oxygen have similar electronegativities, due to the spatial arrangement of atoms.
How does the spatial arrangement of atoms affect tronegativity?
The spatial arrangement around an atom affects tronegativity because it influences the accessibility of the atom's orbitals to other bonding atoms. Steric hindrance or electronic effects resulting from the 3D structure can alter the atom's ability to attract electrons. This means tronegativity influence bond formation to a greater extent than electronegativity when the molecule has a complex shape.
What practical benefits does understanding tronegativity provide in chemistry?
Understanding tronegativity can improve our predictions of molecular properties, like reactivity and spectral characteristics. Because tronegativity influence bond formation by incorporating the physical layout of a molecule, scientists can better tailor the synthesis of molecules with specific functions based on a more nuanced understanding of bond polarity and electron distribution.
So, next time you're wondering why molecules stick together the way they do, remember the subtle but powerful influence of tronegativity. It's just one piece of the puzzle, but understanding how tronegativity influence bond formation? helps us predict and explain the incredible diversity of the chemical world. Pretty neat, right?