Explain Electrical Conductivity of Sodium Chloride
Sodium chloride, commonly known as table salt, exhibits a fascinating duality in its electrical behavior, transitioning from an insulator in its solid-state to a conductor when dissolved in water or heated to a molten state. This transformation is fundamentally linked to its ionic structure, where sodium (Na) atoms transfer electrons to chlorine (Cl) atoms, forming positively charged sodium ions (Na+) and negatively charged chloride ions (Cl-). The mobility of these ions, heavily researched within the field of electrochemistry, is significantly constrained within the rigid crystal lattice of solid NaCl, hindering charge transport and thus preventing electrical conductivity. However, the introduction of a polar solvent like water, investigated extensively in laboratories specializing in material science, or the application of sufficient thermal energy to induce melting disrupts this lattice, liberating the ions and enabling them to carry charge, thereby enabling us to explain the electrical conductivity of sodium chloride under these conditions.
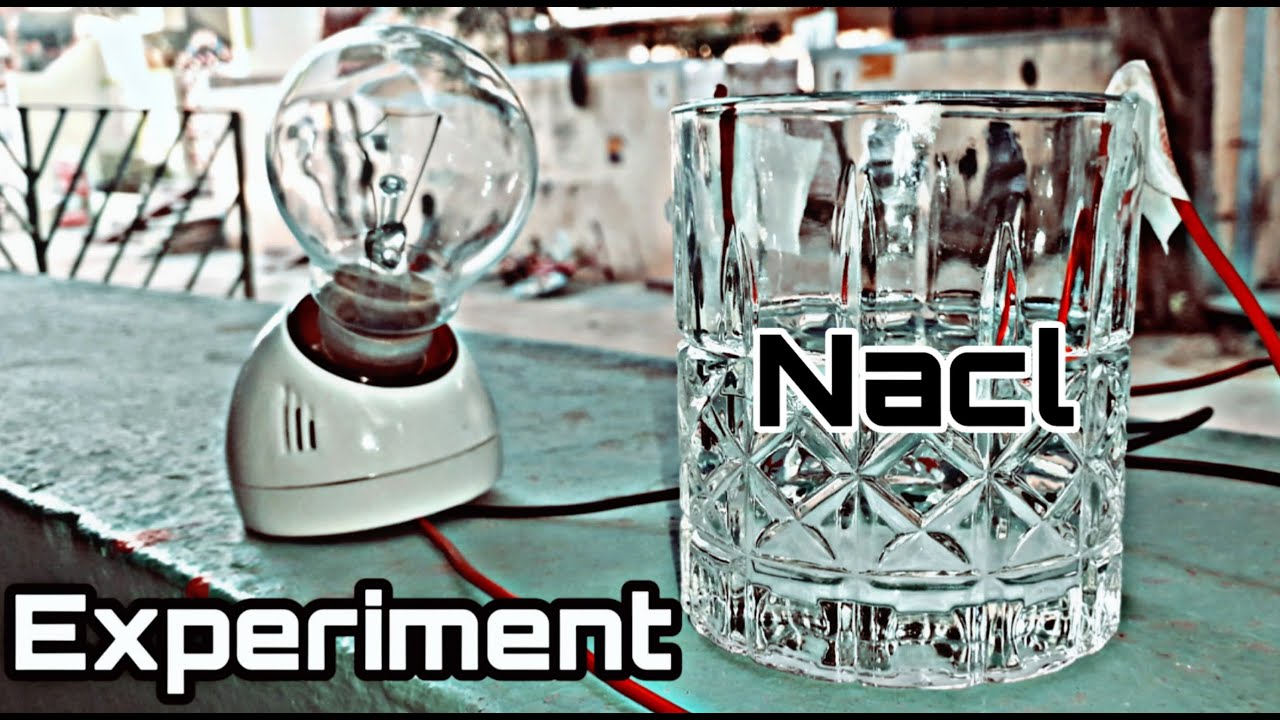
Image taken from the YouTube channel The TechNical Boy , from the video titled Experiment! Electrical Conductivity in Sodium Chloride .
Electrical conductivity, at its core, is the measure of a material's ability to conduct electric current. This phenomenon is fundamentally linked to the presence and mobility of charged particles within the material.
In aqueous solutions, electrical conductivity hinges on the presence of ions – atoms or molecules that have gained or lost electrons, carrying a net electrical charge. These ions act as charge carriers, facilitating the flow of electric current through the solution.
The Role of Electrolytes: Sodium Chloride as a Prime Example
Substances that dissociate into ions when dissolved in water are known as electrolytes. Sodium chloride (NaCl), common table salt, serves as a quintessential example.
When NaCl dissolves, it breaks apart into positively charged sodium ions (Na+) and negatively charged chloride ions (Cl-). These ions, dispersed throughout the water, render the solution capable of conducting electricity.
The higher the concentration of these ions, the greater the solution's conductivity. This direct relationship underscores the crucial role electrolytes play in enabling electrical conduction in aqueous environments.
Solid vs. Solution: A Tale of Two Conductivities
A stark contrast exists between the electrical conductivity of solid NaCl and its aqueous solution. Solid NaCl, in its crystalline form, is a poor conductor of electricity.
This is because the ions are locked in a rigid lattice structure, held together by strong ionic bonds. Consequently, the ions lack the freedom to move and carry charge.
However, when NaCl dissolves in water, these ionic bonds are disrupted. The individual Na+ and Cl- ions are liberated and become mobile.
This newfound mobility is the key to the dramatic increase in electrical conductivity observed in saltwater solutions. The ability of ions to move freely under the influence of an electric field is what distinguishes a conductive solution from a non-conductive solid.
Electrical conductivity, at its core, is the measure of a material's ability to conduct electric current. This phenomenon is fundamentally linked to the presence and mobility of charged particles within the material.
In aqueous solutions, electrical conductivity hinges on the presence of ions – atoms or molecules that have gained or lost electrons, carrying a net electrical charge. These ions act as charge carriers, facilitating the flow of electric current through the solution.
The Dissolution Process: How Salt Turns into Ions
Having established the foundational role of ions in electrical conductivity, we now turn to the critical process by which sodium chloride, or common salt, dissolves in water to create these charge-carrying ions. This process is essential for understanding why saltwater conducts electricity while solid salt does not.
The dissolution of NaCl is a complex interplay of electrostatic forces and molecular interactions, culminating in the separation of the salt crystal into individual, solvated ions.
Unveiling the Dissociation of Sodium Chloride
At the heart of saltwater conductivity lies the dissociation of sodium chloride (NaCl) into its constituent ions: sodium (Na+) and chloride (Cl-). When crystalline NaCl is introduced into water, the polar water molecules begin to interact with the ions on the surface of the crystal.
These interactions are driven by electrostatic attraction: the slightly negative oxygen atoms in water are attracted to the positively charged Na+ ions, and the slightly positive hydrogen atoms are attracted to the negatively charged Cl- ions.
As more water molecules cluster around the surface ions, the ionic bonds holding the NaCl crystal together are weakened. Eventually, the energy from the water molecules overcomes the lattice energy of the crystal, and the ions begin to break away.
This process results in the liberation of individual Na+ and Cl- ions, which are now free to move within the aqueous solution.
Solvation: Water's Role in Ion Stabilization
While dissociation is the initial step, the solvation of these ions by water molecules is equally critical for maintaining conductivity. Solvation refers to the process by which solvent molecules (in this case, water) surround and stabilize solute ions.
Each Na+ and Cl- ion becomes surrounded by a sphere of water molecules, oriented with their dipoles aligned to maximize electrostatic attraction. The positive sodium ions are surrounded by water molecules with their oxygen atoms facing inward, while the negative chloride ions are surrounded by water molecules with their hydrogen atoms oriented towards the chloride ion.
This arrangement effectively shields the ions from each other, preventing them from recombining and reforming the NaCl crystal.
More importantly, solvation stabilizes the ions in solution. The energy released during solvation compensates for the energy required to break the ionic bonds in the crystal lattice. This energetic stabilization is crucial for maintaining a high concentration of mobile ions, thereby ensuring high electrical conductivity.
Without solvation, the dissociated ions would quickly recombine, rendering the solution non-conductive.
The solvation process also enhances ion mobility. While the solvated ions are larger and more massive than bare ions, the water molecules surrounding them reduce the electrostatic interactions with other ions in the solution. This reduction in interionic forces allows the solvated ions to move more freely under the influence of an electric field, contributing significantly to the solution's conductivity.
Therefore, both the dissociation and subsequent solvation of ions are indispensable for the electrical conduction of a sodium chloride solution. It is the dynamic interplay between water molecules and ions that transforms solid salt into a conductive solution, highlighting the profound influence of molecular interactions on macroscopic electrical properties.
Electrical conductivity, at its core, is the measure of a material's ability to conduct electric current. This phenomenon is fundamentally linked to the presence and mobility of charged particles within the material.
In aqueous solutions, electrical conductivity hinges on the presence of ions – atoms or molecules that have gained or lost electrons, carrying a net electrical charge. These ions act as charge carriers, facilitating the flow of electric current through the solution.
Factors Influencing Conductivity in Saltwater
Having established the foundational role of ions in electrical conductivity, we now turn our attention to the factors that govern the extent to which saltwater conducts electricity. While the presence of ions is a prerequisite, the efficiency of conduction is influenced by a complex interplay of variables, from the intrinsic properties of the ions themselves to the external conditions of the solution.
Ion Mobility and its Determinants
Ion mobility, defined as the velocity of an ion in an electric field, is a critical determinant of electrical conductivity. The greater the mobility of the ions, the more effectively they can transport charge through the solution.
However, ion mobility is not a static property. Several factors can influence it significantly:
- Ion Size: Smaller ions generally exhibit higher mobility due to reduced hydrodynamic drag. Larger ions experience greater resistance as they move through the solvent.
- Ion Charge: Ions with higher charges experience a greater electrostatic force from the applied electric field, leading to increased mobility. However, higher charge can also lead to stronger interactions with solvent molecules, which might reduce mobility to some extent.
- Solvent Viscosity: The viscosity of the solvent (in this case, water) directly impacts ion mobility. A more viscous solvent provides greater resistance to ion movement, reducing conductivity. Temperature plays a crucial role here, as increasing temperature lowers viscosity, therefore increasing conductivity.
The relationship between these factors is complex, and the overall effect on conductivity depends on their interplay.
The Electric Field and Current Generation
An electric field is the driving force behind the movement of ions in a solution, and hence, the generation of electric current. When an electric field is applied across a saltwater solution, the positively charged sodium ions (Na+) are attracted to the negatively charged electrode (cathode), while the negatively charged chloride ions (Cl-) migrate towards the positively charged electrode (anode).
This directional movement of ions constitutes an electric current. The magnitude of the current is directly proportional to both the strength of the electric field and the concentration of mobile ions in the solution.
Ohm's Law and Electrolytic Resistance
Ohm's Law, expressed as V = IR, provides a fundamental relationship between voltage (V), current (I), and resistance (R) in a circuit. In the context of saltwater solutions, Ohm's Law remains applicable, but the nature of resistance in electrolytic solutions warrants closer examination.
Unlike metallic conductors where resistance arises from electron scattering, resistance in electrolytic solutions stems from:
- Interionic Interactions: The electrostatic interactions between ions impede their movement, contributing to resistance.
- Ion-Solvent Interactions: The solvation of ions increases their effective size and hydrodynamic drag, further hindering their mobility and increasing resistance.
- Electrode Polarization: The build-up of ions at the electrode surfaces can create a counter-electromotive force, effectively increasing resistance.
Understanding these factors is key to predicting and controlling the electrical behavior of saltwater solutions.
Resistivity and Conductivity: An Inverse Relationship
Resistivity (ρ) is an intrinsic material property that quantifies its opposition to the flow of electric current. It is the inverse of conductivity (σ):
σ = 1/ρ
A material with high resistivity is a poor conductor, while a material with low resistivity is a good conductor. In the context of saltwater, resistivity depends on the concentration of ions, their mobility, and the temperature of the solution.
Therefore, by understanding the factors that influence resistivity, we can effectively modulate and control the conductivity of saltwater solutions, optimizing their performance in a range of applications.
Electrical conductivity, at its core, is the measure of a material's ability to conduct electric current. This phenomenon is fundamentally linked to the presence and mobility of charged particles within the material.
In aqueous solutions, electrical conductivity hinges on the presence of ions – atoms or molecules that have gained or lost electrons, carrying a net electrical charge. These ions act as charge carriers, facilitating the flow of electric current through the solution.
Measuring Conductivity: An Experimental Approach
Having explored the underlying principles that govern the electrical conductivity of saltwater, we now shift our focus to the practical aspects of measuring this property. Experimental determination of conductivity is crucial for a wide range of applications, from environmental monitoring to industrial process control. This section will detail the instrumentation and techniques employed to accurately quantify the conductivity of aqueous solutions.
The Role of Electrodes in Conductivity Measurement
Electrodes are indispensable components in any experimental setup designed to measure the electrical conductivity of a solution. They serve as the interface between the electronic measuring instrument and the ionic solution, facilitating the transfer of charge that enables the measurement.
Typically, conductivity measurements utilize two or four electrodes immersed in the solution. The electrodes are constructed from a conductive material, often platinum or stainless steel, chosen for their inertness and resistance to corrosion in aqueous environments.
In a two-electrode system, an alternating voltage is applied across the electrodes, and the resulting current is measured. The ratio of voltage to current yields the resistance of the solution between the electrodes.
Four-electrode systems, on the other hand, employ a more sophisticated configuration. Two electrodes are used to apply the current, while the other two are used to measure the potential difference across a defined volume of the solution. This configuration minimizes the effects of electrode polarization, leading to more accurate measurements, especially in solutions with higher conductivity.
Conductivity Meters: Tools for Quantitative Analysis
A conductivity meter, also known as a conductometer, is the instrument used to measure the electrical conductivity of a solution. Modern conductivity meters are sophisticated electronic devices that provide direct readings of conductivity, often with temperature compensation features for accurate results.
These meters operate by applying an alternating current (AC) to the electrodes immersed in the solution. The AC signal is used to prevent polarization at the electrodes, which would otherwise introduce errors in the measurement.
The meter measures the resulting current and calculates the conductance (G), which is the inverse of resistance (R):
G = 1/R
Conductivity (σ) is then calculated from conductance using the cell constant (k), which is a geometrical factor that accounts for the electrode spacing and surface area:
σ = k * G
The cell constant is determined by calibrating the conductivity meter with a solution of known conductivity, such as a standard potassium chloride (KCl) solution. Calibration ensures the accuracy and reliability of the measurements.
Types of Conductivity Meters
Different types of conductivity meters are available, each suited for specific applications:
-
Benchtop Meters: These are laboratory-grade instruments designed for precise and accurate measurements. They often feature advanced functionalities such as data logging and connectivity options.
-
Portable Meters: These compact and rugged meters are designed for field measurements. They are battery-powered and often waterproof, making them suitable for environmental monitoring and on-site testing.
-
Online Meters: These meters are designed for continuous monitoring of conductivity in industrial processes. They are typically installed directly into pipelines or tanks and provide real-time data for process control.
Measurement Considerations
Several factors can affect the accuracy of conductivity measurements:
-
Temperature: Conductivity is temperature-dependent, with higher temperatures generally leading to higher conductivity. Conductivity meters often incorporate temperature compensation to normalize measurements to a standard temperature (e.g., 25°C).
-
Electrode Fouling: Contamination of the electrodes can affect their performance and accuracy. Regular cleaning and maintenance of the electrodes are essential.
-
Air Bubbles: Air bubbles trapped on the electrode surfaces can impede the flow of current and lead to errors. Gently agitating the solution or using electrodes with smooth surfaces can help minimize this issue.
By carefully considering these factors and utilizing appropriate instrumentation, accurate and reliable measurements of electrical conductivity can be obtained, providing valuable insights into the properties of ionic solutions.
Experimental observations are paramount in understanding any physical phenomenon; however, a comprehensive understanding necessitates delving into the theoretical frameworks that explain why these phenomena occur. In the case of electrolytic conductivity, empirical measurements are augmented by established theoretical models that provide a deeper insight into the behavior of ionic solutions.
This section explores the theoretical underpinnings of electrolytic conductivity, including Arrhenius's theory of electrolytic dissociation and the concept of ionic strength. These concepts are instrumental in comprehending the principles governing the behavior of conductive solutions.
Theoretical Models: Unveiling the Principles of Saltwater Conductivity
While experimentation provides valuable data on the conductivity of saltwater, theoretical models are essential for a more profound understanding of why saltwater conducts electricity in the manner observed. These models offer a framework to predict and explain the behavior of ions in solution, enabling us to move beyond mere observation to true comprehension.
Arrhenius's Theory of Electrolytic Dissociation: A Foundation for Understanding
Svante Arrhenius's theory of electrolytic dissociation, proposed in the late 19th century, marked a paradigm shift in understanding the behavior of electrolytes in solution. This theory posits that electrolytes, such as sodium chloride, dissociate into ions when dissolved in water.
Prior to Arrhenius's work, it was widely believed that ions were formed only during the process of electrolysis. Arrhenius proposed that ions exist independently in solution, even in the absence of an applied electric field. This groundbreaking idea revolutionized the understanding of electrolytic conductivity.
According to Arrhenius, the degree of dissociation, represented by α, indicates the fraction of electrolyte molecules that have dissociated into ions. A strong electrolyte, like NaCl, dissociates almost completely (α ≈ 1) in dilute solutions, meaning that nearly all NaCl molecules break apart into Na+ and Cl- ions.
This complete or near-complete dissociation is the primary reason for the high conductivity of saltwater. The presence of a high concentration of mobile ions facilitates the efficient transport of electric charge through the solution.
Limitations of Arrhenius's Theory
While Arrhenius's theory was revolutionary, it is not without its limitations. The theory assumes that ions behave independently in solution and does not account for interionic interactions. In concentrated solutions, these interactions become significant and can affect the conductivity of the solution.
Furthermore, Arrhenius's theory does not explicitly address the role of the solvent (water) in the dissociation process. Modern theories incorporate the concept of solvation, recognizing that water molecules play a crucial role in stabilizing ions in solution.
Ionic Strength: Quantifying the Influence of Ion Concentration
Ionic strength is a measure of the total concentration of ions in a solution. It takes into account both the concentration and the charge of each ion present. Ionic strength is a crucial parameter that influences the behavior of ions in solution and, consequently, the electrical conductivity.
The ionic strength (I) of a solution is defined as:
I = 1/2 Σ(cᵢzᵢ²)
Where cᵢ is the molar concentration of ion i, and zᵢ is the charge number of that ion. The summation is taken over all ions in the solution.
Solutions with high ionic strength exhibit complex ion-ion interactions, which can significantly affect the conductivity. These interactions can reduce the mobility of ions, leading to a decrease in conductivity compared to what would be expected based solely on the concentration of the electrolyte.
The Debye-Hückel Theory: Accounting for Interionic Interactions
The Debye-Hückel theory provides a more sophisticated treatment of electrolytic solutions by accounting for interionic interactions. This theory proposes that each ion in solution is surrounded by an "ionic atmosphere" of oppositely charged ions.
This ionic atmosphere effectively screens the charge of the central ion, reducing its ability to conduct electricity. The Debye-Hückel theory predicts that the conductivity of a solution will decrease with increasing ionic strength due to the increased screening effect.
While the Debye-Hückel theory provides a more accurate description of electrolytic solutions than Arrhenius's theory, it is still an approximation and is most accurate for dilute solutions. At high concentrations, more complex models are required to accurately predict the behavior of ions in solution.
In summary, theoretical models such as Arrhenius's theory and the concept of ionic strength provide a framework for understanding the fundamental principles governing the electrical conductivity of saltwater. These models, while not perfect, offer valuable insights into the behavior of ions in solution and enable us to predict and explain the conductivity of electrolytic solutions.
Real-World Applications and Implications of Saltwater Conductivity
The principles governing saltwater conductivity, seemingly confined to laboratory experiments, resonate profoundly across diverse fields. From the assessment of environmental health to the intricacies of biological processes and the efficiency of industrial operations, the ability of saltwater to conduct electricity serves as a critical parameter and a powerful analytical tool. Understanding these applications is not merely academic; it illuminates the tangible impact of fundamental scientific concepts on our daily lives.
Environmental Monitoring: Assessing Water Quality Through Salinity
The conductivity of saltwater is intrinsically linked to its salinity, the concentration of dissolved salts. This relationship forms the basis for salinity monitoring, a crucial aspect of environmental science. Elevated salinity levels in freshwater sources can indicate pollution from agricultural runoff, industrial discharge, or saltwater intrusion, all of which pose significant threats to aquatic ecosystems and human water supplies.
By continuously monitoring conductivity, scientists and environmental agencies can detect changes in salinity, identify potential sources of contamination, and implement timely mitigation strategies. Conductivity measurements serve as an early warning system, alerting us to imbalances in aquatic environments before they escalate into irreversible damage.
Moreover, conductivity measurements are used to assess the health of coastal estuaries, delicate ecosystems where freshwater and saltwater mix. Changes in salinity within these zones can disrupt the balance of the local flora and fauna, affecting biodiversity and the overall productivity of these vital habitats. Accurate salinity monitoring is therefore indispensable for the effective management and conservation of coastal resources.
Biological Systems: The Electrochemical Basis of Life
The phenomenon of saltwater conductivity extends far beyond environmental science, playing a pivotal role in the functioning of biological systems. Nerve impulse transmission, the very foundation of our sensory perception, movement, and thought processes, relies on the flow of ions across cell membranes. These ions, primarily sodium (Na+) and potassium (K+), create electrochemical gradients that drive the transmission of electrical signals along nerve fibers.
The human body is essentially a complex network of electrolytic solutions. The controlled movement of ions through these solutions is the basis for neural communication, muscle contraction, and a host of other essential functions. Disruptions in ion balance, whether due to disease, dehydration, or electrolyte imbalances, can severely impair these processes, leading to a range of neurological and physiological disorders.
The study of bioelectricity and its relationship to ionic conductivity has led to significant advancements in medicine. From electrocardiograms (ECGs) that monitor heart activity to electroencephalograms (EEGs) that record brain activity, diagnostic tools rely on the principles of ionic conduction to provide insights into the health and function of various organ systems.
Industrial Applications: Water Treatment and Beyond
The conductivity of saltwater also finds practical applications in various industrial processes, most notably in water treatment. Conductivity measurements are used to monitor the effectiveness of desalination plants, which remove salt from seawater to produce potable water.
Regular monitoring of the conductivity of the treated water ensures that it meets the required purity standards and is safe for consumption. Similarly, in wastewater treatment plants, conductivity measurements can be used to assess the levels of dissolved salts and other contaminants, helping to optimize the treatment process and ensure compliance with environmental regulations.
Beyond water treatment, conductivity measurements are also used in a variety of other industrial applications, including the production of chemicals, the processing of food, and the manufacturing of electronic components. In these contexts, conductivity serves as a critical process parameter, enabling manufacturers to control product quality, optimize efficiency, and minimize waste.
Video: Explain Electrical Conductivity of Sodium Chloride
FAQs: Electrical Conductivity of Sodium Chloride
Why doesn't solid sodium chloride conduct electricity?
Solid sodium chloride (NaCl), or table salt, doesn't conduct electricity because its ions (Na+ and Cl-) are locked in a rigid crystal lattice. These ions are not free to move and carry charge, which is necessary to explain the electrical conductivity of sodium chloride in this state.
How does sodium chloride conduct electricity when dissolved in water?
When sodium chloride is dissolved in water, it dissociates into free-moving sodium (Na+) and chloride (Cl-) ions. These ions can now move freely throughout the solution. This mobility allows them to carry an electrical charge, which explains the electrical conductivity of sodium chloride in aqueous solution.
Does molten (liquid) sodium chloride conduct electricity?
Yes, molten sodium chloride conducts electricity. When heated to a high temperature, the rigid crystal lattice breaks down, and the sodium (Na+) and chloride (Cl-) ions become mobile. These mobile ions are then free to carry charge, thus explaining the electrical conductivity of sodium chloride in its molten state.
Is the electrical conductivity of sodium chloride affected by temperature?
Yes, the electrical conductivity of sodium chloride solutions is affected by temperature. Higher temperatures generally lead to greater ion mobility. This increased mobility results in a higher electrical current when a voltage is applied, thereby impacting and helping to explain the electrical conductivity of sodium chloride.
So, next time you're sprinkling salt on your fries, remember there's a whole world of electrical wizardry going on inside that little crystal! Hopefully, this clears up how explain the electrical conductivity of sodium chloride works, and you can impress your friends with your newfound knowledge of ionic compounds and their amazing (sometimes) ability to conduct electricity!