Bohr Model of Sodium: Electron Configuration Simplified
The Bohr model of sodium, a cornerstone in understanding atomic structure, simplifies the electron configuration of sodium atoms, illustrating how electrons occupy specific energy levels or shells around the nucleus, akin to planets orbiting the sun, as first proposed by Niels Bohr. These shells, labeled K, L, and M, can be visualized using the quantum numbers to represent the discrete energy states electrons occupy within the sodium atom, while the periodic table organizes elements like sodium based on their electron configurations, which ultimately dictate their chemical properties. The model explains the characteristic spectra of sodium, which arises from electrons transitioning between these energy levels, emitting or absorbing photons of specific wavelengths.
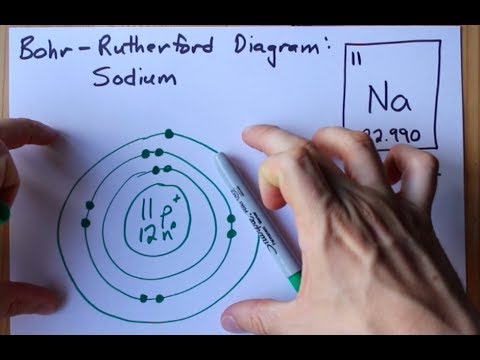
Image taken from the YouTube channel chemistNATE , from the video titled How to Draw the Bohr-Rutherford Diagram of Sodium .
Unveiling the Bohr Model and Atomic Structure: A Crucial Leap in Understanding the Atom
The Bohr model, proposed by Niels Bohr in 1913, represents a watershed moment in the history of atomic theory. It marked a significant departure from earlier, less successful models, providing a framework for understanding the behavior of electrons within atoms and paving the way for the development of modern quantum mechanics.
The model's conceptual simplicity, combined with its ability to explain observed phenomena, quickly established it as a cornerstone of atomic physics. While later superseded by more sophisticated theories, the Bohr model's influence remains undeniable, and its concepts continue to be valuable tools for visualizing and understanding atomic structure.
The Significance of the Bohr Model
The Bohr model was not merely an incremental improvement; it was a paradigm shift. Prior models, like the "plum pudding" model and even Rutherford's nuclear model, struggled to reconcile theoretical predictions with experimental observations, particularly concerning the discrete nature of atomic spectra.
Bohr's postulates, which incorporated quantum concepts, offered a revolutionary explanation for these spectra, positioning the model as the first to successfully predict certain atomic properties. The model provided a tangible framework for understanding electron behavior, specifically the concept that electrons orbit the nucleus in specific, quantized energy levels, which were a huge leap from classical physics.
Moving Beyond Previous Limitations
Prior to Bohr, models of the atom were plagued by inconsistencies and failures. Rutherford's model, while correctly positing a dense, positively charged nucleus, faced a critical challenge: according to classical electromagnetism, electrons orbiting the nucleus should continuously radiate energy, causing them to spiral into the nucleus and rendering atoms unstable.
The Bohr model elegantly addressed this paradox by postulating that electrons can only exist in specific, quantized orbits, preventing them from continuously radiating energy. Furthermore, it was built upon the quantum theory of Max Planck, which revolutionized the understanding of energy itself. These orbits corresponded to distinct energy levels.
Impact on Understanding Electron Behavior
Perhaps the most significant contribution of the Bohr model was its introduction of the concept of quantized energy levels for electrons. This groundbreaking idea explained how electrons can only occupy specific orbits with defined energy, and can jump from one orbit to another, emitting or absorbing energy.
This simple, yet profound idea provided a framework for understanding electron behavior. It also opened up new avenues for exploring the relationship between atomic structure and chemical properties. By incorporating Planck's quantum theory, Bohr linked the atom's structure to the fundamental nature of energy itself.
Exploring Key Concepts, Evidence, and Legacy
This article aims to delve into the core principles of the Bohr model. We will explore the evidence that supported its development, and assess its legacy in shaping our current understanding of atomic physics.
By examining its key concepts and its historical context, we will gain a deeper appreciation for the Bohr model's enduring significance in the world of science. We will also see the model in action using an example element, solidifying the basic principles and how they can be applied.
Why We Needed Bohr: Addressing the Shortcomings of Earlier Models
The Bohr model, proposed by Niels Bohr in 1913, represents a watershed moment in the history of atomic theory. It marked a significant departure from earlier, less successful models, providing a framework for understanding the behavior of electrons within atoms. Before Bohr, the atomic landscape was riddled with inconsistencies and unexplained phenomena, particularly concerning the nature of atomic spectra. Let's delve into the reasons why the Bohr model became an essential breakthrough.
The Flaws of the Rutherford Model
Ernest Rutherford's model, while revolutionary in its own right, presented a critical problem: the stability of the atom. Rutherford proposed that electrons orbited the nucleus much like planets orbit the sun.
However, according to classical physics, an accelerating charged particle (such as an electron orbiting the nucleus) should continuously emit electromagnetic radiation.
This would cause the electron to lose energy, spiraling into the nucleus and leading to the atom's inevitable collapse. Rutherford's model simply couldn't explain why atoms are stable.
The Mystery of Atomic Spectra
Another significant challenge faced by earlier models was their inability to account for the discrete nature of atomic spectra. When elements are heated or energized, they emit light at specific wavelengths, creating a unique line spectrum for each element.
These spectral lines are not continuous; rather, they appear as distinct, narrow bands of color. Classical physics could not explain why atoms emit light only at these specific wavelengths, and not across the entire spectrum.
This quantization of light emission was a major puzzle that needed to be solved.
Bohr's Solution: Quantized Energy Levels
The Bohr model offered a radical solution to these problems by introducing the concept of quantized energy levels. Bohr proposed that electrons could only exist in specific, allowed orbits around the nucleus, each corresponding to a distinct energy level.
This meant that electrons could not continuously lose or gain energy while orbiting; they could only transition between these allowed energy levels.
When an electron jumps from a higher energy level to a lower one, it emits a photon of light with a specific energy (and therefore, wavelength) equal to the energy difference between the two levels.
This explained the discrete nature of atomic spectra, as only photons with specific energies could be emitted.
Addressing Atomic Stability
Bohr's postulates also addressed the issue of atomic stability. By proposing that electrons could only occupy specific energy levels, he essentially stated that electrons in these allowed orbits did not radiate energy.
This contradicted classical physics, but it provided a way to explain why electrons did not spiral into the nucleus. The Bohr model, therefore, offered a framework for understanding atomic stability that was missing in previous models.
The Impact of Bohr's Model
The Bohr model was a crucial stepping stone in the development of modern quantum mechanics. While it had its own limitations and was eventually superseded by more sophisticated models, it provided the first successful explanation for the quantization of energy levels and the discrete nature of atomic spectra.
It revolutionized our understanding of atomic structure and paved the way for future advancements in the field. By addressing the shortcomings of earlier models, Bohr's work laid the groundwork for the quantum mechanical model of the atom, which is still used today.
Key Concepts: Demystifying the Bohr Model's Principles
The Bohr model, proposed by Niels Bohr in 1913, represents a watershed moment in the history of atomic theory. It marked a significant departure from earlier, less successful models, providing a framework for understanding the behavior of electrons within atoms. Before delving into its practical applications, it's crucial to grasp the fundamental principles upon which the Bohr model rests. These key concepts, including energy levels, quantization, electron configuration, ground and excited states, photon emission, and the role of valence electrons, form the bedrock of this revolutionary model.
Energy Levels (Electron Shells)
One of the cornerstone concepts of the Bohr model is the idea that electrons do not orbit the nucleus in just any path. Instead, they reside in specific, quantized energy levels, often visualized as electron shells. These shells are designated by the principal quantum number, n, which can be any positive integer (n = 1, 2, 3, etc.). The higher the n value, the farther the shell is from the nucleus and the greater the energy level.
Each shell has a limited capacity for holding electrons. The first shell (n=1) can hold a maximum of two electrons, the second shell (n=2) can hold a maximum of eight, and so on. This concept is critical to understanding electron configuration and how atoms interact with each other.
Quantization of Energy
Classical physics suggested that energy could be emitted or absorbed in continuous amounts. The Bohr model, however, introduced the groundbreaking idea that energy is absorbed or emitted in discrete packets called quanta. This quantization of energy means that electrons can only exist at specific, defined energy levels within the atom.
An electron cannot possess an energy value between these allowed levels. This postulate directly contradicted classical physics and was essential for explaining the discrete nature of atomic spectra. An electron must absorb a specific amount of energy to move from one energy level to the next.
Electron Configuration
Electron configuration describes the arrangement of electrons within the different energy levels and sublevels of an atom. Understanding electron configuration is crucial for predicting an atom's chemical behavior. For instance, knowing how many electrons occupy the outermost shell is critical for understanding its bonding behavior.
Electron configuration diagrams are visual tools that help represent this arrangement. These diagrams typically show the nucleus, the electron shells, and the number of electrons occupying each shell. They provide a simple way to visualize the electronic structure of an atom and understand its properties.
Ground State and Excited State
An atom is said to be in its ground state when all its electrons occupy the lowest possible energy levels. This represents the most stable configuration for the atom. However, if an atom absorbs energy (e.g., from heat or light), one or more electrons can jump to higher energy levels, resulting in an excited state.
The excited state is unstable. The electron will tend to return to a lower energy level. This transition is fundamental to how the Bohr model explains spectral lines.
Photon Emission
When an electron transitions from an excited state to a lower energy level, it releases the excess energy in the form of a photon. The energy of the emitted photon is precisely equal to the difference in energy between the two energy levels. This is represented by the equation: E = hf, where E is the energy of the photon, h is Planck's constant, and f is the frequency of the emitted radiation.
The atomic spectra that are observed are a direct consequence of these photon emissions. Each spectral line corresponds to a specific electron transition between energy levels. Analyzing these spectral lines provides valuable information about the energy levels within an atom.
Valence Electrons
Valence electrons are the electrons in the outermost electron shell of an atom. These electrons are primarily responsible for the chemical properties of an atom. They determine how an atom will interact with other atoms to form chemical bonds.
The number of valence electrons dictates an atom's reactivity and bonding behavior. Atoms with a full outer shell (like noble gases) are generally unreactive, while atoms with incomplete outer shells tend to form bonds to achieve a stable configuration. Understanding valence electrons is essential for predicting how atoms will combine to form molecules.
Niels Bohr: The Architect of the Model
The Bohr model, proposed by Niels Bohr in 1913, represents a watershed moment in the history of atomic theory. It marked a significant departure from earlier, less successful models, providing a framework for understanding the behavior of electrons within atoms. Before delving into its practical applications, it's crucial to understand the intellectual journey and the driving forces behind its creator, Niels Bohr.
A Glimpse into Bohr's Life and Scientific Journey
Niels Henrik David Bohr, born in Copenhagen in 1885, was immersed in an intellectual environment from an early age. His father, Christian Bohr, was a professor of physiology, and his upbringing instilled in him a deep appreciation for scientific inquiry.
Bohr earned his doctorate in physics from the University of Copenhagen in 1911. He then went on to work with J.J. Thomson and Ernest Rutherford in England. These experiences profoundly shaped his understanding of atomic structure and the limitations of existing models.
The Drive Behind the Model: Addressing Scientific Inconsistencies
Bohr's motivation stemmed from a deep dissatisfaction with the existing models of the atom, primarily Rutherford's model. While Rutherford's model correctly posited a nuclear atom with electrons orbiting a central nucleus, it failed to explain certain key observations.
Classical physics predicted that electrons orbiting the nucleus would continuously radiate energy, spiraling into the nucleus and causing the atom to collapse. This, of course, didn't happen.
Furthermore, Rutherford's model couldn't account for the discrete spectral lines observed when elements were heated or exposed to electricity. These spectral lines indicated that atoms emitted light at specific, well-defined wavelengths, a phenomenon that classical physics couldn't explain. Bohr recognized these inconsistencies as critical flaws. He sought to develop a model that could resolve these issues and provide a more accurate description of atomic behavior.
Influences and Collaborations: Building on the Shoulders of Giants
Bohr's work was not conducted in isolation; he drew inspiration from and collaborated with several prominent scientists of his time.
Ernest Rutherford: The Nuclear Foundation
Bohr's time working with Ernest Rutherford was pivotal. Rutherford's discovery of the atomic nucleus provided the foundation upon which Bohr built his model. Bohr adopted Rutherford's nuclear model but sought to improve it by incorporating quantum concepts.
Johannes Rydberg: The Spectral Connection
Johannes Rydberg's work on atomic spectra, particularly the Rydberg formula, was another key influence. The Rydberg formula described the wavelengths of light emitted by hydrogen atoms. Bohr's model sought to provide a theoretical explanation for the empirical observations captured by the Rydberg formula.
Max Planck and Albert Einstein: Quantum Leap
Bohr was heavily influenced by the groundbreaking work of Max Planck and Albert Einstein on quantum theory. Planck's concept of quantized energy and Einstein's explanation of the photoelectric effect provided the theoretical tools necessary for Bohr to develop his model. He incorporated the idea of energy quantization, which was groundbreaking at the time, stating that electrons could only occupy specific energy levels.
Bohr's brilliance lay in his ability to synthesize these diverse influences and create a coherent model of the atom that addressed the shortcomings of earlier theories. His model, though later superseded by more advanced quantum mechanical models, provided a crucial stepping stone in our understanding of the atomic world.
Evidence in Action: Supporting the Bohr Model Through Atomic Spectra
The Bohr model, proposed by Niels Bohr in 1913, represents a watershed moment in the history of atomic theory. It marked a significant departure from earlier, less successful models, providing a framework for understanding the behavior of electrons within atoms. Before delving into its practical applications, it's essential to consider the evidence that initially supported and validated this groundbreaking model, with a primary focus on its ability to explain atomic spectra.
Deciphering the Language of Light: Atomic Spectra
Atomic spectra, the patterns of light emitted or absorbed by atoms, served as a crucial piece of evidence in solidifying the Bohr model's validity. These spectra are unique "fingerprints" for each element, characterized by discrete lines at specific wavelengths, a phenomenon that defied explanation by classical physics.
The Bohr model offered a revolutionary explanation, positing that these lines corresponded to specific electron transitions between quantized energy levels.
Quantized Jumps and Spectral Lines
According to the Bohr model, electrons can only occupy certain energy levels within an atom. When an electron transitions from a higher energy level to a lower one, it emits a photon of light with an energy precisely equal to the difference between those levels.
This energy difference determines the wavelength (and thus the color) of the emitted light. Because the energy levels are quantized, only specific wavelengths are emitted, resulting in the discrete spectral lines observed.
The frequency of the light (and thus the colour) is directly proportional to the amount of energy released as the electron falls from one energy level to another.
Spectroscopy: Unveiling Atomic Secrets
Spectroscopy, the study of the interaction between matter and electromagnetic radiation, played a pivotal role in confirming the quantization of energy levels predicted by the Bohr model. By analyzing the wavelengths of light emitted or absorbed by substances, spectroscopists could precisely determine the energy differences between electron orbitals within atoms.
The technique allowed scientists to not only verify the existence of discrete energy levels but also to measure them with high accuracy. This confirmation lent strong support to the core principles of the Bohr model.
The Rydberg Formula: A Mathematical Validation
Even before Bohr’s model was fully developed, the Rydberg formula (developed by Johannes Rydberg) provided a mathematical relationship that accurately predicted the wavelengths of spectral lines for hydrogen. The Bohr model provided a theoretical basis for this formula, linking it to the fundamental constants and the quantization of energy. This connection further strengthened the model’s credibility and its ability to quantitatively describe atomic phenomena.
Limitations and Further Refinements
While the Bohr model successfully explained the hydrogen atom's spectrum and provided a crucial stepping stone in understanding atomic structure, it's essential to acknowledge its limitations.
It could not accurately predict the spectra of more complex atoms with multiple electrons, and it lacked a theoretical explanation for the intensities of spectral lines.
These shortcomings paved the way for the development of more sophisticated quantum mechanical models. Still, the Bohr model remains a cornerstone of atomic theory, demonstrating the power of quantization in explaining the behavior of matter at the atomic level.
Bohr in Practice: Applying the Model to Sodium (Z=11)
The Bohr model, proposed by Niels Bohr in 1913, represents a watershed moment in the history of atomic theory. It marked a significant departure from earlier, less successful models, providing a framework for understanding the behavior of electrons within atoms. Before delving into more complex elements, it's instructive to see how the Bohr model operates in a practical example. Here, we will apply the Bohr model to the element Sodium (Na), which has an atomic number of 11 (Z=11), illustrating its utility in determining electron configurations.
Deciphering Sodium's Electron Configuration
Sodium, with its atomic number of 11, possesses 11 protons in its nucleus. In a neutral atom, the number of electrons is equal to the number of protons; therefore, Sodium also has 11 electrons. The Bohr model dictates that these electrons occupy specific energy levels, or shells, surrounding the nucleus.
The first shell (n=1), closest to the nucleus, can hold a maximum of 2 electrons. The second shell (n=2) can accommodate up to 8 electrons. The third shell (n=3) can hold up to 18 electrons, but in the case of Sodium, it will not be fully occupied.
Following these rules, we can distribute Sodium's 11 electrons as follows:
- First Shell (n=1): 2 electrons
- Second Shell (n=2): 8 electrons
- Third Shell (n=3): 1 electron
This distribution, denoted as 2-8-1, represents Sodium's electron configuration according to the Bohr model.
Visualizing Sodium: The Bohr Diagram
A Bohr diagram provides a visual representation of the electron configuration. It consists of a central nucleus, represented by the element symbol (Na) or the number of protons. Concentric circles represent the electron shells, with electrons depicted as dots or crosses orbiting the nucleus.
For Sodium, the Bohr diagram would show:
- A central nucleus labeled "Na" or "11+."
- A first shell with 2 electrons.
- A second shell with 8 electrons.
- A third shell with 1 electron.
This visual aid effectively illustrates the arrangement of electrons within the Sodium atom, as predicted by the Bohr model.
The Lone Electron: Valence and Reactivity
The single electron in Sodium's outermost shell (n=3) is of particular importance. This electron is called a valence electron. Valence electrons dictate an atom's chemical behavior and its tendency to form chemical bonds with other atoms. Sodium's single valence electron makes it highly reactive.
Core Electrons: The Inner Shield
While valence electrons are the key players in chemical reactions, the electrons in the inner shells (n=1 and n=2) are known as core electrons. These electrons are tightly bound to the nucleus and are not typically involved in chemical bonding. They provide a "shield" for the nucleus and contribute to the overall stability of the atom.
Core Electrons: The Inner Circle
The Bohr model, while a simplified representation of atomic structure, provides a useful framework for understanding the roles of different electrons within an atom. Having explored how the model applies to valence electrons, it's equally important to consider the electrons residing closer to the nucleus: the core electrons.
These electrons, unlike their valence counterparts, play a less direct role in chemical bonding and reactivity. Understanding their behavior and influence is crucial for a comprehensive view of atomic behavior.
Defining Core Electrons
Core electrons are the electrons that occupy the innermost energy levels, or shells, of an atom. They are strongly attracted to the positively charged nucleus and are shielded from external influences by the valence electrons.
These electrons are found in the lower energy levels, closest to the nucleus. This means they are tightly bound to the atom and require a significant amount of energy to be removed or excited.
Essentially, they are all the electrons that are not valence electrons. For elements in the first few rows of the periodic table, identifying core electrons is straightforward. However, with heavier elements and the inclusion of d and f orbitals, identifying and understanding the behavior of core electrons can become more complex.
Location and Shielding Effect
The location of core electrons close to the nucleus is significant for several reasons. Their proximity results in a strong electrostatic attraction, effectively stabilizing the atom.
Furthermore, core electrons play a role in shielding the valence electrons from the full positive charge of the nucleus. This shielding effect reduces the effective nuclear charge experienced by the valence electrons, influencing their energy levels and reactivity.
The greater the number of core electrons, the greater the shielding effect, and the weaker the attraction experienced by valence electrons. This is a critical factor in determining an element's ionization energy and electronegativity.
Limited Role in Chemical Reactions
The primary distinction between core and valence electrons lies in their participation in chemical reactions. Core electrons are generally considered inert and do not participate in the formation of chemical bonds.
Their tight binding to the nucleus makes them energetically unavailable for interactions with other atoms. The energy required to remove or excite a core electron is typically far greater than the energy available in most chemical reactions.
Instead, valence electrons, which are located in the outermost shell and are less tightly bound, are the key players in chemical bonding. These are the electrons that interact with other atoms, forming molecules and compounds.
Exceptions and Considerations
While core electrons are generally inert, there are exceptions. In some cases, particularly with transition metals and heavier elements, core electrons can exhibit some influence on chemical behavior. This often arises from relativistic effects or incomplete shielding.
However, for the vast majority of chemical reactions involving lighter elements, the core electrons remain spectators. Their primary role is to stabilize the atom and contribute to the overall electron configuration, indirectly influencing the behavior of valence electrons.
Understanding the distinction between core and valence electrons is fundamental to comprehending chemical bonding and reactivity. The Bohr model, despite its limitations, offers a conceptual framework for appreciating this critical difference.
Video: Bohr Model of Sodium: Electron Configuration Simplified
FAQs: Bohr Model of Sodium
How does the Bohr model represent sodium's electron configuration?
The Bohr model of sodium shows its 11 electrons arranged in specific energy levels or shells around the nucleus. Two electrons occupy the innermost shell (n=1), eight occupy the second shell (n=2), and one single electron resides in the outermost shell (n=3).
Why is only the outermost electron in the Bohr model of sodium important for chemical bonding?
The outermost electron, also called the valence electron, is key because it's involved in chemical reactions. Sodium readily donates this electron to achieve a stable, filled outer shell like the noble gases. This is a core concept when understanding the bohr model of sodium.
What are the limitations of using the Bohr model to describe sodium's electrons?
The Bohr model simplifies the arrangement of electrons. It doesn't accurately depict the complex shapes of electron orbitals or the probability of finding electrons in specific regions, issues better addressed by quantum mechanics. The bohr model of sodium is therefore more of a visual aid than a fully accurate representation.
How does the Bohr model of sodium help us understand its reactivity?
The Bohr model of sodium clearly shows its one valence electron. This highlights sodium's tendency to lose that electron and form a positive ion (Na+). This loss easily explains why sodium is a highly reactive metal, as depicted well through understanding the bohr model of sodium.
So, next time you're pondering the periodic table or just need a quick refresher on how atoms are structured, remember the Bohr model of sodium. It's a simplified yet surprisingly helpful way to visualize those electrons whizzing around and understand why sodium behaves the way it does!