Bohr Model of Oxygen: Atomic Structure Explained
The Bohr model of oxygen, a foundational concept in chemistry, provides a simplified yet insightful representation of the oxygen atom's structure. Niels Bohr, the pioneering physicist, developed this model based on earlier work by Ernest Rutherford, particularly Rutherford's model of the atom, to address shortcomings in classical physics when applied to atomic structure. The oxygen atom, as depicted by the Bohr model of oxygen, features eight electrons arranged in distinct energy levels, or shells, orbiting the nucleus, a concept that contrasted sharply with earlier plum pudding models. Understanding the Bohr model of oxygen is a crucial stepping stone for comprehending more advanced concepts in quantum mechanics and molecular bonding.
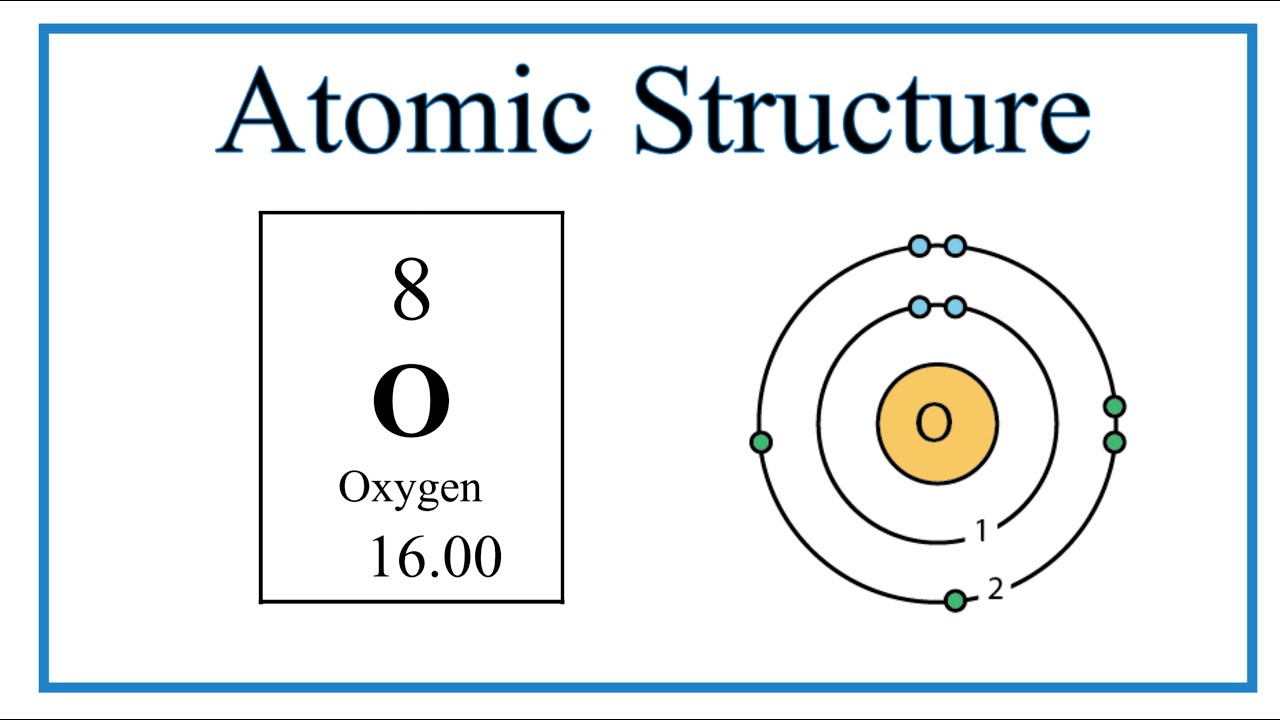
Image taken from the YouTube channel Wayne Breslyn (Dr. B.) , from the video titled Atomic Structure (Bohr Model) for Oxygen (O) .
A Quantum Leap: Introducing the Bohr Model
The Bohr model, conceived by Niels Bohr in 1913, represents a pivotal stepping stone in our journey to comprehend atomic structure. It wasn't the final destination, but rather a crucial landmark that irrevocably altered the landscape of atomic theory. This model provided a more refined understanding of how atoms are structured, challenging earlier notions and setting the stage for future quantum mechanical models.
The Pre-Bohr Atomic Landscape
Before Bohr, the prevailing model was that of Ernest Rutherford, which proposed a nuclear atom with electrons orbiting a central nucleus, similar to planets orbiting the sun. This model, while groundbreaking, presented significant problems rooted in classical physics.
Specifically, according to classical electromagnetism, an accelerating charged particle, like an orbiting electron, should continuously emit electromagnetic radiation.
This would cause the electron to lose energy and spiral into the nucleus, resulting in an unstable atom — a clear contradiction to the observed stability of matter. Rutherford’s model failed to explain why atoms were stable.
Quantization: A Revolutionary Concept
Bohr addressed these shortcomings by introducing the concept of quantized energy levels. He postulated that electrons could only occupy specific orbits around the nucleus, each corresponding to a discrete energy level.
Electrons could jump between these energy levels by absorbing or emitting energy in the form of photons, but they could not exist between these defined states. This quantization of energy levels was a radical departure from classical physics, which allowed for continuous energy values.
This single idea provided a framework that began to explain the stability of atoms and the discrete spectral lines observed in atomic emissions.
Niels Bohr: Architect of the Atom
Niels Bohr was a Danish physicist whose work fundamentally reshaped our understanding of the atom. His integration of quantum theory with classical physics to explain atomic structure earned him the Nobel Prize in Physics in 1922.
Bohr’s model, while eventually superseded by more sophisticated quantum mechanical models, remains a cornerstone of atomic theory. It elegantly introduced the concept of quantized energy levels and provided a visualizable, albeit simplified, picture of atomic structure that is still invaluable in teaching the basics of chemistry and physics today. Bohr’s work set the stage for the quantum revolution, paving the way for future discoveries.
Building the Foundation: Key Influences on Bohr's Atomic Model
The Bohr model, conceived by Niels Bohr in 1913, represents a pivotal stepping stone in our journey to comprehend atomic structure. It wasn't the final destination, but rather a crucial landmark that irrevocably altered the landscape of atomic theory. This model provided a more refined understanding of how electrons are arranged within atoms and how they interact with energy. Its development was not born in isolation, but rather built upon the groundwork laid by prior scientific discoveries and concepts.
Refining Rutherford's Vision
Ernest Rutherford's model, which depicted the atom as a miniature solar system with electrons orbiting a central nucleus, was revolutionary for its time. However, it had a critical flaw: according to classical physics, these orbiting electrons should continuously radiate energy, causing them to spiral into the nucleus and rendering atoms unstable. This prediction contradicted the observed stability of matter.
Bohr directly addressed this issue by incorporating the emerging principles of quantum mechanics. He retained the core structure of Rutherford's model but proposed that electrons could only occupy specific, quantized energy levels, thus preventing the continuous energy loss predicted by classical physics. This strategic modification was pivotal for the model’s success.
Planck's Quantum Theory: Quantizing Energy
Central to Bohr's model was the incorporation of Max Planck's quantum theory. Planck, while studying blackbody radiation, discovered that energy is not emitted or absorbed continuously, but rather in discrete packets called quanta. This revolutionary concept challenged the long-held tenets of classical physics.
Planck's Constant
The relationship between the energy of a quantum (E) and its frequency (ν) is defined by the equation E = hν, where h is Planck's constant (approximately 6.626 x 10-34 Js). This constant represents the fundamental unit of quantization and plays a vital role in numerous quantum phenomena.
Challenging Classical Physics
Planck's theory shattered the classical notion that energy could take on any continuous value. By suggesting that energy is quantized, Planck laid the foundation for a new understanding of the microscopic world. This radical departure from classical physics was essential for Bohr's model, as it provided a mechanism for quantizing electron energy levels within the atom.
Rydberg's Formula: Predicting Spectral Lines
Another key influence on Bohr's model was Johannes Rydberg's formula, an empirical equation that accurately predicted the wavelengths of light emitted by hydrogen. Rydberg's formula provided compelling evidence that energy levels within atoms are quantized, even before Bohr developed his theoretical framework.
The Rydberg Constant
Rydberg's formula is expressed as: 1/λ = R (1/n12 - 1/n22), where λ is the wavelength of the emitted light, R is the Rydberg constant (approximately 1.097 x 107 m-1), and n1 and n2 are integers representing the initial and final energy levels of the electron. The Rydberg constant is an experimentally determined value that directly reflects the quantized nature of energy transitions in hydrogen.
Relating the Formula to Observed Spectral Lines
The elegance of Rydberg's formula lies in its ability to precisely predict the spectral lines observed in the hydrogen emission spectrum. Each spectral line corresponds to a specific electron transition between two energy levels. By plugging in different values for n1 and n2, one can accurately calculate the wavelength of the emitted light, confirming the quantized nature of electron energy levels and validating the formula's predictive power. This formula helped Bohr formulate the principles of electron transitions.
Core Principles of the Bohr Model: A Step-by-Step Breakdown
Building upon the groundwork laid by Planck and Rydberg, Bohr crafted his atomic model, which, at its core, postulates a specific architecture for the atom and dictates how electrons behave within it. To truly grasp the significance of Bohr's contribution, it's essential to dissect its fundamental tenets.
The Atomic Foundation: Protons, Neutrons, and Electrons
At the heart of the Bohr model lies the basic understanding of atomic structure: the atom is composed of protons, neutrons, and electrons.
Protons, carrying a positive charge, and neutrons, being electrically neutral, reside within the atom's nucleus.
Electrons, negatively charged particles, orbit the nucleus much like planets around a star.
The nucleus, therefore, acts as the dense, positively charged center of the atom, exerting an attractive force on the orbiting electrons.
Quantized Orbits: Energy Levels and Shells
A revolutionary aspect of the Bohr model is the concept of quantized energy levels.
Electrons, according to Bohr, can only occupy specific orbits or shells around the nucleus, each corresponding to a distinct energy level.
These energy levels are quantized, meaning electrons cannot exist between these defined orbits.
These levels are numbered, starting with n=1, the energy level closest to the nucleus, often referred to as the ground state. n=2, n=3, and so on, represent successively higher energy levels that are farther from the nucleus. The higher the number, the higher the energy and the greater the distance from the nucleus.
Electronic Configuration: Arranging Electrons in Shells
The electron configuration describes how electrons are arranged within these energy levels or shells. There are rules governing how these shells are filled.
The first shell (n=1) can hold a maximum of two electrons.
The second shell (n=2) can accommodate up to eight electrons.
The third shell (n=3) can hold a maximum of 18 electrons, and so on. This can be calculated using the formula 2n2, where 'n' is the principal quantum number representing the energy level.
For example, Hydrogen (H) with only one electron, has an electron configuration of 1s1 (one electron in the first shell). Helium (He) with two electrons, has a configuration of 1s2 (two electrons, completely filling the first shell).
Electron Transitions: Excitation and Relaxation
Electrons are not static within their energy levels; they can transition between them. When an atom absorbs energy (e.g., heat or light), an electron can jump from a lower energy level to a higher energy level. This process is known as excitation.
Conversely, when an electron drops from a higher energy level to a lower energy level, it releases energy in the form of a photon. This is relaxation.
Photons: The Messengers of Energy
A photon is a particle of light (electromagnetic radiation) that carries energy.
The energy of the photon emitted or absorbed during an electron transition is precisely equal to the difference in energy between the two energy levels. This relationship is defined by the equation E = hf, where E is the energy, h is Planck's constant, and f is the frequency of the photon.
The frequency (and thus the wavelength) of the emitted photon determines its position on the electromagnetic spectrum. This relationship explains the origin of spectral lines.
Each element has a unique set of spectral lines, acting like a fingerprint and allowing scientists to identify the element. These spectral lines are direct evidence of photon emission during electron transitions.
Ground State and Excited State: Defining Atomic Energy
The ground state represents the lowest energy state an atom can exist in. In this state, all electrons occupy the lowest possible energy levels.
When an atom absorbs energy, one or more electrons jump to higher energy levels, resulting in an excited state. This state is unstable, and the electron(s) will quickly return to their ground state, releasing energy in the process.
Oxygen Under the Microscope: Applying the Bohr Model to a Real Element
Building upon the groundwork laid by Planck and Rydberg, Bohr crafted his atomic model, which, at its core, postulates a specific architecture for the atom and dictates how electrons behave within it. To truly grasp the significance of Bohr's contribution, it's essential to dissect its functionality by applying it to a real-world element. Oxygen, a cornerstone of life and a ubiquitous element in the universe, serves as an excellent case study for visualizing and understanding the Bohr model in action.
Analyzing Oxygen Through the Bohr Lens
Oxygen (O), with its atomic number of 8, offers a relatively simple yet illustrative example for applying the Bohr model. Understanding its composition is paramount.
A neutral oxygen atom contains 8 protons within its nucleus, defining it as oxygen. It also possesses 8 electrons orbiting the nucleus to balance the positive charge of the protons, maintaining electrical neutrality.
While the number of neutrons can vary (leading to isotopes), the most common isotope of oxygen, Oxygen-16, has 8 neutrons.
According to the Bohr model, these electrons occupy specific energy levels or shells. A visual representation of the Bohr model for Oxygen would depict the nucleus containing 8 protons and typically 8 neutrons, surrounded by two electron shells.
The first shell, closest to the nucleus (K-shell), can hold a maximum of two electrons, while the second shell (L-shell) can hold up to eight.
Electron Configuration of Oxygen: Filling the Shells
The electron configuration of Oxygen, according to the Bohr model, reveals much about its chemical behavior. The 8 electrons are distributed as follows: 2 electrons in the innermost K-shell and 6 electrons in the outer L-shell.
This can be represented as 1s² 2s² 2p⁴ in more modern notation, but the Bohr model simplifies this to two shells with the specified electron counts.
A crucial detail highlighted by the Bohr model is the presence of two unpaired electrons in the outer L-shell. These unpaired electrons make oxygen highly reactive, as it strives to achieve a stable, filled outer shell (octet) by forming chemical bonds with other elements.
This drive to complete its octet dictates much of oxygen's chemistry.
Electron Transitions and Photon Emissions in Oxygen
The Bohr model elucidates how electron transitions lead to the emission of photons, which manifest as characteristic spectral lines. While the Bohr model provides a simplified explanation, it captures the essence of the process.
Imagine an electron in the L-shell of Oxygen absorbing energy, such as from heat or light. This causes the electron to jump to a higher, unstable energy level further from the nucleus.
Almost instantaneously, the electron will fall back to its original energy level (or to another lower level).
As it does so, it releases the excess energy in the form of a photon. The energy of this photon corresponds precisely to the difference in energy between the two electron shells.
This specific energy translates to a particular wavelength of light, which can be observed as a spectral line.
The characteristic spectral lines of oxygen are a direct result of these electron transitions. Each element has a unique set of spectral lines, acting as a fingerprint that identifies the element. Analyzing these spectral lines allows scientists to determine the elemental composition of various materials, including distant stars and nebulae.
Decoding Light: Spectroscopy and the Electromagnetic Spectrum
[Oxygen Under the Microscope: Applying the Bohr Model to a Real Element Building upon the groundwork laid by Planck and Rydberg, Bohr crafted his atomic model, which, at its core, postulates a specific architecture for the atom and dictates how electrons behave within it. To truly grasp the significance of Bohr's contribution, it's essential to discuss the tools and concepts used to experimentally validate and interpret the model, specifically spectroscopy and its relationship to the electromagnetic spectrum.]
Spectroscopy offers a powerful means to investigate the internal structure of atoms by analyzing the light they emit or absorb. This section explores the fundamental principles of spectroscopy and demonstrates how it connects electron transitions within the atom to the observable patterns of light across the electromagnetic spectrum.
Spectroscopy as a Tool for Atomic Exploration
At its core, spectroscopy is the study of the interaction between matter and electromagnetic radiation. It provides a method to identify the composition and properties of substances by analyzing their unique spectral signatures.
Spectroscopy hinges on the principle that atoms absorb or emit light at specific wavelengths, corresponding to the energy differences between their quantized energy levels.
Different spectroscopic techniques exist, each optimized for different regions of the electromagnetic spectrum and specific types of analysis.
Emission Spectroscopy
Emission spectroscopy involves heating a sample until it emits light. The emitted light is then passed through a prism or diffraction grating to separate it into its constituent wavelengths, producing an emission spectrum. The pattern of bright lines in the emission spectrum is unique to each element, acting as a fingerprint for identification.
Absorption Spectroscopy
Conversely, absorption spectroscopy involves passing a beam of light through a sample and measuring the wavelengths that are absorbed. The resulting absorption spectrum shows dark lines at wavelengths that correspond to the energy required to promote electrons to higher energy levels.
The Electromagnetic Spectrum and Atomic Signatures
Electron transitions within an atom, as described by the Bohr model, directly correlate to the emission or absorption of photons. Each photon possesses a specific energy, which dictates its position within the electromagnetic spectrum.
Energy and Wavelength
The energy of a photon is inversely proportional to its wavelength. High-energy photons, such as those in the ultraviolet (UV) region, have short wavelengths, while low-energy photons, such as those in the infrared (IR) region, have longer wavelengths.
Spectral Fingerprints Across the Spectrum
When an electron transitions from a higher energy level to a lower energy level, it emits a photon with a specific wavelength. These photons create distinct lines in the emission spectrum. The position of these lines within the electromagnetic spectrum (UV, visible, IR, etc.) depends on the magnitude of the energy difference between the electron's initial and final states.
Similarly, in absorption spectroscopy, the wavelengths of light that an atom absorbs reveal the specific energy transitions that are possible within that atom.
Observing Oxygen's Light with a Spectroscope
A spectroscope is an instrument used to observe and analyze the spectrum of light emitted or absorbed by a substance. By examining the spectral lines produced by oxygen (or other elements), we can gain insights into its atomic structure.
How a Spectroscope Works
In essence, a spectroscope separates light into its constituent wavelengths, allowing us to visualize the spectrum. Light enters the spectroscope through a narrow slit and is then dispersed by a prism or diffraction grating.
This separates the light into its different wavelengths, which are then projected onto a screen or detector.
Oxygen's Spectral Signature
When oxygen is excited, such as in a gas discharge tube, it emits light with a characteristic set of spectral lines. These lines appear as distinct bands of color when viewed through a spectroscope. The wavelengths of these lines are specific to oxygen and can be used to identify its presence in a sample.
The specific pattern of these spectral lines provides compelling evidence for the quantized energy levels predicted by the Bohr model. The precise wavelengths of light emitted directly reflect the energy differences between allowed electron orbits within the oxygen atom. These lines, therefore, provide tangible proof of the fundamental principles underpinning Bohr's revolutionary atomic model.
Quantum Leap: The Bohr Model and Quantum Mechanics
Building upon the groundwork laid by Planck and Rydberg, Bohr crafted his atomic model, which, at its core, postulates a specific architecture for the atom and dictates how electrons behave within it. To truly grasp the Bohr model's significance, we must examine its relationship with the broader, more sophisticated framework of quantum mechanics.
The Quantum Underpinnings of the Bohr Model
The Bohr model, in many ways, represents an early foray into applying quantum principles to the structure of the atom.
Prior to Bohr, classical physics reigned supreme, but it failed to adequately explain atomic phenomena like discrete spectral lines.
Bohr's genius lay in recognizing the need for a new paradigm.
Quantized Energy Levels: A Revolutionary Concept
The most crucial contribution of the Bohr model was introducing the concept of quantized energy levels.
Electrons, according to Bohr, could only occupy specific, discrete orbits around the nucleus, each corresponding to a particular energy level.
This idea, directly inspired by Planck's quantum theory, was a radical departure from classical physics, which allowed for a continuous range of energy values.
This quantization elegantly explained the discrete nature of atomic spectra, as electrons could only absorb or emit energy in specific amounts when transitioning between these levels.
Limitations in Light of Modern Quantum Mechanics
While the Bohr model was a monumental step forward, it is essential to acknowledge its limitations in light of modern quantum mechanics/quantum theory.
As our understanding of the atom has deepened, the Bohr model has been superseded by more comprehensive and accurate descriptions.
Applicability to Simple Systems
One of the most significant limitations is that the Bohr model only provides accurate predictions for hydrogen and other single-electron ions (like He+).
It fails spectacularly when applied to atoms with multiple electrons due to its inability to account for electron-electron interactions.
The Absence of Electron Spin and Wave-Particle Duality
The Bohr model treats electrons as point-like particles orbiting the nucleus in well-defined paths.
This is a simplification that ignores the wave-particle duality of electrons, a fundamental concept in quantum mechanics.
Furthermore, the Bohr model does not incorporate electron spin, an intrinsic property of electrons that significantly influences atomic behavior and chemical bonding.
The Schrödinger Equation and the Quantum Mechanical Model
The development of the Schrödinger equation marked a turning point in our understanding of atomic structure.
This equation, a cornerstone of quantum mechanics, provides a mathematical framework for describing the behavior of electrons in atoms.
The solutions to the Schrödinger equation yield atomic orbitals, which are three-dimensional regions of space where an electron is most likely to be found.
This quantum mechanical model paints a far more nuanced and accurate picture of the atom than the Bohr model, accounting for electron-electron interactions, electron spin, and the wave-like nature of electrons.
In conclusion, while the Bohr model has been superseded by more sophisticated theories, it represents a crucial historical milestone. It pioneered the application of quantum principles to atomic structure, laying the foundation for our modern understanding of the atom. Its simplicity makes it a valuable tool for introductory education, even as we recognize its limitations in the context of contemporary quantum mechanics.
The Outermost Electrons: Valence and Chemical Reactivity
Building upon the groundwork laid by Planck and Rydberg, Bohr crafted his atomic model, which, at its core, postulates a specific architecture for the atom and dictates how electrons behave within it. To truly grasp the Bohr model's significance, we must examine its relationship with the broader, more chemically relevant concept of valence electrons and reactivity. The Bohr model, while simplified, provides a fundamental understanding of how these outermost electrons dictate an element's interactions with the world around it.
Valence Electrons: The Key to Chemical Properties
Valence electrons are defined as the electrons residing in the outermost electron shell of an atom. These are the electrons that participate in chemical bonding, determining how atoms interact to form molecules and compounds.
The number and arrangement of valence electrons dictate an element's characteristic chemical behavior. Understanding this behavior is crucial for predicting how elements will combine and react under various conditions.
The Octet Rule and Chemical Stability
The driving force behind chemical bonding is the tendency of atoms to achieve a stable electron configuration, typically resembling that of a noble gas. This stability is often achieved when an atom has eight electrons in its valence shell, fulfilling what is known as the octet rule.
Atoms may achieve this stable octet by gaining, losing, or sharing electrons with other atoms, leading to the formation of chemical bonds. The Bohr model helps visualize how electrons are exchanged to achieve this stability.
Oxygen's Reactivity: A Valence Electron Perspective
Oxygen (O), with its atomic number of 8, offers an excellent example to illustrate the significance of valence electrons. An oxygen atom possesses six electrons in its outermost shell.
This electron configuration means that oxygen requires two additional electrons to complete its octet, making it highly reactive. Oxygen readily forms chemical bonds to achieve this stable configuration.
Oxygen's Bonding Behavior
Oxygen's strong desire to gain two electrons results in its ability to form a variety of chemical bonds. It can form:
- Covalent bonds by sharing electrons with other nonmetal atoms.
- Ionic bonds by accepting electrons from metal atoms.
This versatile bonding capability is why oxygen is found in countless compounds essential to life and industrial processes.
Water and Oxides: Essential Oxygen Compounds
Two of the most important classes of compounds involving oxygen are water (H₂O) and oxides. In water, oxygen shares electrons with two hydrogen atoms, forming covalent bonds. The resulting polar molecule is essential for all known life.
Oxides, formed through the reaction of oxygen with other elements, are also ubiquitous. Metal oxides, such as iron oxide (rust), and nonmetal oxides, such as carbon dioxide (CO₂), play significant roles in geological processes, industrial applications, and environmental chemistry.
The Importance of Water
Water's unique properties, stemming from oxygen's bonding, sustain life on Earth.
Its polarity allows it to act as a universal solvent, facilitating countless biochemical reactions.
The Roles of Oxides
Oxides play key roles in:
- Geology: Forming the basis of many minerals and rocks.
- Industry: Used as catalysts, pigments, and building materials.
- Environment: Participating in essential cycles like the carbon cycle.
While the Bohr model has limitations, it provides a foundational understanding of valence electrons and how they govern chemical reactivity. By understanding the principles of the Bohr model, one can appreciate the crucial role of oxygen's six valence electrons in its diverse chemical interactions and the formation of life-sustaining compounds. The concept of the valence electron remains a vital aspect of chemistry, underpinning our understanding of how matter interacts and forms the world around us.
Video: Bohr Model of Oxygen: Atomic Structure Explained
Frequently Asked Questions
How many electrons are in the Bohr model of oxygen and where are they located?
The Bohr model of oxygen depicts oxygen with 8 electrons. Two electrons reside in the first energy level (closest to the nucleus) and six electrons occupy the second energy level.
Does the Bohr model of oxygen accurately represent the real structure of an oxygen atom?
The Bohr model is a simplified representation. While useful for understanding basic atomic structure, it doesn't fully reflect the complex electron behavior described by quantum mechanics. The bohr model of oxygen gives a general idea but isn't perfect.
What does the nucleus of oxygen contain according to the Bohr model?
The Bohr model of oxygen shows the nucleus containing 8 protons and typically 8 neutrons. The number of protons defines the element, while the number of neutrons can vary creating different isotopes of oxygen.
How can the bohr model of oxygen help me understand how oxygen reacts with other elements?
The Bohr model of oxygen helps visualize oxygen's six valence electrons (outermost electrons). These electrons are crucial in forming chemical bonds with other elements, driving oxygen's reactivity.
So, there you have it! Hopefully, this breaks down the Bohr model of oxygen in a way that makes sense. It’s a simplified picture, for sure, but it’s a great stepping stone to understanding the more complex world of atomic structure. Keep exploring, and who knows, maybe you'll be the one refining our understanding of atoms next!