ATP vs ADP: Energy Currency (Adenosine Triphosphate)
Adenosine triphosphate (ATP), a fundamental component in cellular energy transfer, operates within the intricate biochemical pathways of all known life forms, from the simplest bacteria to complex multicellular organisms. The mitochondria, often regarded as the powerhouses of the cell, orchestrate ATP synthesis through oxidative phosphorylation. The reversible interconversion of adenosine triphosphate v adp is vital, as the hydrolysis of ATP to adenosine diphosphate (ADP) releases energy that drives numerous cellular processes. Renowned biochemist Fritz Albert Lipmann's work elucidated the pivotal role of ATP in energy metabolism. Researchers at the National Institutes of Health (NIH) continue to investigate the complexities surrounding ATP and ADP, seeking to further understand their implications in health and disease.
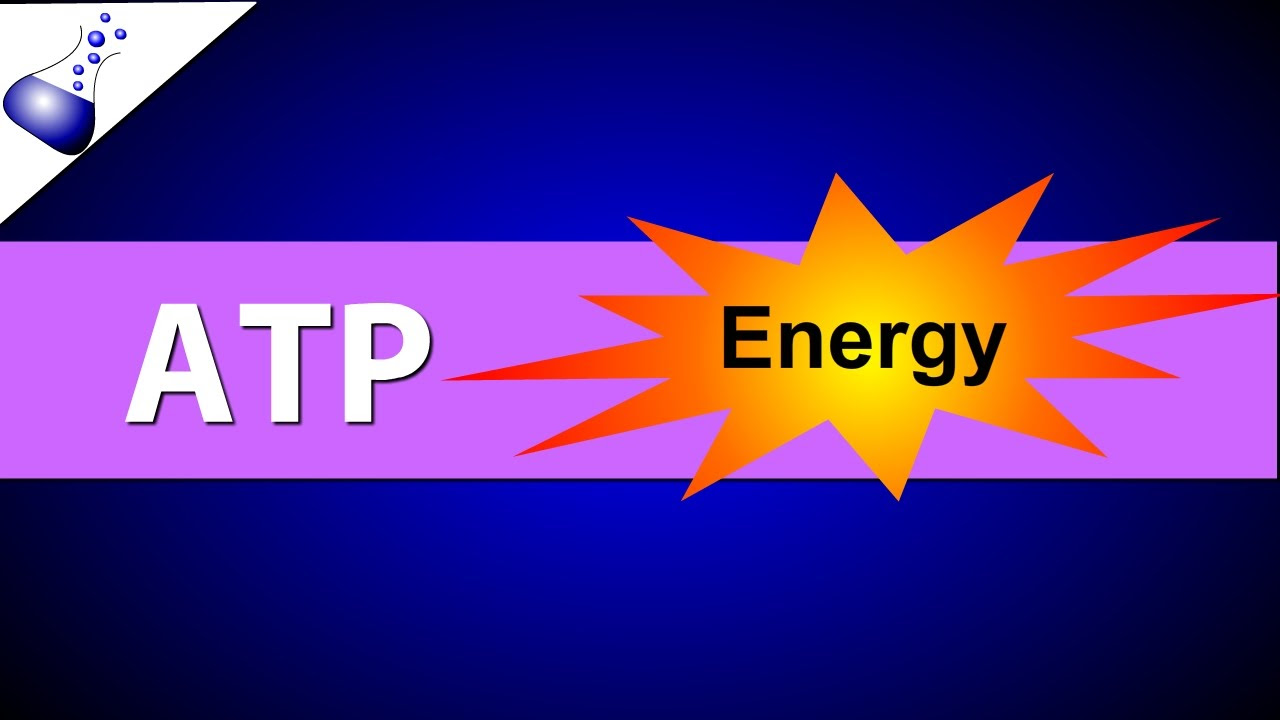
Image taken from the YouTube channel RicochetScience , from the video titled ATP (Adenosine Triphosphate) .
The Essence of Life: ATP - The Cell's Universal Energy Currency
Adenosine Triphosphate, or ATP, stands as the primary energy currency within the intricate world of cellular biology. It is the molecule that fuels virtually all life processes, from muscle contraction to nerve impulse transmission and protein synthesis. Without ATP, cells would cease to function, and life as we know it would be impossible.
Decoding the Molecular Structure of ATP
At its core, ATP comprises an adenosine molecule, which itself is composed of adenine (a nitrogenous base) and ribose (a five-carbon sugar). Linked to the ribose sugar are three phosphate groups. These phosphate groups are sequentially attached and are critical to ATP's role as an energy carrier.
The bonds connecting these phosphate groups are termed phosphoanhydride bonds, also known as "high-energy bonds" due to the significant amount of energy released when they are hydrolyzed. It is the breaking of these bonds that provides the energy required for cellular work.
ATP: Powering the Machinery of Life
ATP's pivotal role extends to almost every biological process. It fuels muscle contraction, enabling movement. It powers active transport, allowing cells to import essential molecules and export waste against concentration gradients.
Furthermore, ATP serves as a key substrate in signal transduction pathways, acting as a phosphate donor to activate or deactivate proteins, thereby regulating cellular communication and responses to external stimuli. The utilization of ATP is ubiquitous and indispensable.
A Glimpse into History: The Discovery of ATP
The discovery of ATP is attributed to Karl Lohmann in 1929, who first isolated it from muscle tissue. His initial findings laid the foundation for understanding its role in energy transfer.
Later, Fritz Lipmann further elucidated ATP's central role in cellular metabolism, proposing it as the primary energy currency of the cell. Lipmann's work, particularly his concept of ATP as a common intermediate in energy transfer, earned him the Nobel Prize in Physiology or Medicine in 1953, solidifying ATP's place as one of the most important molecules in biology.
The Structure and Chemistry of ATP: A Molecular Deep Dive
Building upon the understanding of ATP as the cell's energy currency, it becomes imperative to dissect the molecular architecture that underpins its function. This section provides an exhaustive examination of ATP's structural components, focusing on the intricacies of Adenine, Ribose, and the pivotal Phosphate groups. Furthermore, we will elucidate the nature of the high-energy phosphate bonds, their role in energy storage and release, and the dynamic interconversion between ATP, ADP, and AMP, with particular emphasis on the role of Inorganic Phosphate (Pi).
Deconstructing the ATP Molecule: Adenine, Ribose, and Phosphate Groups
ATP, at its core, is a nucleotide, a classification it shares with the building blocks of DNA and RNA. Its molecular structure comprises three fundamental components: Adenine, a nitrogenous base; Ribose, a five-carbon sugar; and a triphosphate group consisting of three Phosphate molecules linked together.
Adenine, a derivative of purine, provides the foundational scaffold for the molecule, lending its chemical identity. Ribose, the pentose sugar, connects to Adenine at the 1' carbon and acts as the bridge to the energetic heart of the molecule - the triphosphate tail.
The triphosphate group, the defining feature of ATP, is a chain of three phosphate groups linked by phosphoanhydride bonds. It is these bonds that are the key to ATP's energy storage capacity.
The High-Energy Phosphate Bonds: A Source of Cellular Power
The phosphoanhydride bonds linking the phosphate groups are commonly referred to as "high-energy" bonds. This designation is not entirely accurate, as the bonds themselves do not possess some special form of stored energy. Instead, the term "high-energy" describes the significant release of free energy that occurs upon their hydrolysis.
The negatively charged phosphate groups repel each other, creating inherent instability within the triphosphate tail. When one of these bonds is broken through hydrolysis (the addition of water), one phosphate group is removed (forming ADP) or two phosphate groups are removed (forming AMP), resulting in a more stable configuration, and energy is released in the process.
This released energy can then be harnessed to drive other cellular reactions that would otherwise be thermodynamically unfavorable.
ATP, ADP, and AMP: A Cycle of Energy Transfer
ATP does not function as a long-term energy storage molecule within the cell. Instead, it acts as an immediate energy donor, constantly being synthesized and broken down to meet the cell's fluctuating energy demands. The interconversion between ATP, Adenosine Diphosphate (ADP), and Adenosine Monophosphate (AMP) represents this dynamic cycle of energy transfer.
The removal of one phosphate group from ATP yields ADP, releasing energy. The removal of a second phosphate group from ADP yields AMP, also releasing energy, though typically to a lesser extent.
Conversely, the addition of a phosphate group to ADP, forming ATP, requires an input of energy, typically derived from cellular respiration or photosynthesis. Similarly, the phosphorylation of AMP to ADP requires energy input.
Inorganic Phosphate (Pi), released during ATP hydrolysis, plays a crucial role in this cycle. It is a critical component in the re-synthesis of ATP from ADP, ensuring the continuous supply of energy to power cellular processes.
ATP Hydrolysis and Energy Coupling: Powering Cellular Reactions
Having established the structural context of ATP, the discussion naturally progresses to the mechanism by which this molecule fuels cellular activities. This section elucidates the process of ATP hydrolysis, the pivotal reaction that unlocks ATP's stored energy. Furthermore, it examines the critical concept of energy coupling, demonstrating how the exergonic hydrolysis of ATP drives endergonic cellular processes. Finally, the enzymatic control of ATP hydrolysis is explored, highlighting the regulatory mechanisms that govern this vital reaction.
ATP Hydrolysis: The Fundamental Energy-Releasing Reaction
ATP hydrolysis, the cleavage of a phosphate bond in ATP, stands as the cornerstone of cellular energy transduction.
This reaction, wherein ATP reacts with water, releases a phosphate group and generates either ADP (adenosine diphosphate) or AMP (adenosine monophosphate), depending on whether one or two phosphate groups are cleaved.
The released energy is not inherent to the phosphate bond itself, but rather due to the change in free energy associated with the reaction, driven by factors such as charge repulsion relief and resonance stabilization of the products.
The equation for ATP hydrolysis to ADP is:
ATP + H2O → ADP + Pi + Energy
Thermodynamics of ATP Hydrolysis: Quantifying Energy Release
The hydrolysis of ATP is an exergonic reaction, meaning it releases energy. This release of energy is quantified by the change in Gibbs Free Energy (ΔG).
Under standard conditions, the ΔG for ATP hydrolysis is approximately -30.5 kJ/mol (-7.3 kcal/mol).
However, the actual ΔG within a cell can vary depending on factors such as temperature, pH, and the concentrations of ATP, ADP, and Pi.
A negative ΔG signifies that the reaction is thermodynamically favorable and proceeds spontaneously, releasing energy that can be harnessed to perform cellular work.
Energy Coupling: Harnessing ATP Hydrolysis to Drive Endergonic Reactions
Cells rarely perform energy-releasing reactions in isolation. Instead, they employ a strategy called energy coupling to link exergonic reactions, such as ATP hydrolysis, with endergonic reactions, which require energy input.
Energy coupling involves using the energy released from ATP hydrolysis to drive an otherwise unfavorable reaction.
A common mechanism for energy coupling involves the phosphorylation of a reactant by ATP.
The phosphate group is transferred from ATP to another molecule, making the subsequent reaction more energetically favorable. This is seen in numerous metabolic pathways and transport processes.
Enzymatic Regulation of ATP Hydrolysis: Fine-Tuning Energy Release
ATP hydrolysis does not occur spontaneously at a significant rate within cells. Enzymes, specifically ATPases, are required to catalyze the reaction and control the rate of ATP hydrolysis.
These enzymes play a crucial role in regulating the availability of energy within cells.
The activity of ATPases can be influenced by a variety of factors, including substrate concentration (ATP, ADP, Pi), pH, and the presence of regulatory molecules.
The regulation of ATP hydrolysis is critical for maintaining cellular homeostasis and ensuring that energy is available when and where it is needed. This precise control allows cells to efficiently manage their energy budget and respond to changing environmental conditions.
ATP Synthesis: Cellular Respiration and Photosynthesis - The Energy Generation Pathways
Having explored the mechanism by which ATP releases energy, it is imperative to investigate the reverse process: ATP synthesis. This section elucidates the two dominant pathways responsible for generating ATP in biological systems: cellular respiration and photosynthesis. The intricacies of each process, along with the pivotal roles of key molecules such as glucose, NAD+/NADH, and FAD/FADH2, will be thoroughly examined.
Cellular Respiration: Harvesting Energy from Organic Molecules
Cellular respiration represents the primary catabolic pathway employed by organisms to extract energy from organic molecules, chiefly glucose. This intricate process comprises a series of interconnected metabolic reactions that culminate in the production of ATP. It is important to note that while glucose is a prime energy source, other molecules like fats and proteins can also be used.
Glycolysis: The Initial Stage of Glucose Breakdown
Glycolysis, the initial stage of cellular respiration, occurs in the cytoplasm and involves the breakdown of glucose (a six-carbon molecule) into two molecules of pyruvate (a three-carbon molecule). This process generates a small amount of ATP and NADH. Though Glycolysis yields some ATP directly, it’s vital role is the preparation of Pyruvate for further ATP generation.
The Krebs Cycle (Citric Acid Cycle): Oxidizing Pyruvate for Energy Carriers
The Krebs Cycle, also known as the Citric Acid Cycle, takes place in the mitochondrial matrix. Here, pyruvate is converted to acetyl-CoA, which then enters the cycle. Through a series of enzymatic reactions, acetyl-CoA is completely oxidized, releasing carbon dioxide, ATP, NADH, and FADH2. The Krebs cycle is critical for capturing high-energy electrons in the form of NADH and FADH2, which will then be used in the electron transport chain.
Electron Transport Chain: Establishing the Proton Gradient
The electron transport chain (ETC) is located in the inner mitochondrial membrane. NADH and FADH2, generated during glycolysis and the Krebs cycle, donate electrons to the ETC. As electrons move through the chain, protons (H+) are pumped from the mitochondrial matrix into the intermembrane space, creating an electrochemical gradient.
Chemiosmosis: Harnessing the Proton Gradient to Drive ATP Synthesis
The proton gradient established by the electron transport chain drives ATP synthesis through a process called chemiosmosis. Protons flow back across the inner mitochondrial membrane through ATP synthase, a transmembrane protein complex. This flow of protons provides the energy for ATP synthase to phosphorylate ADP, producing ATP.
Oxidative Phosphorylation: The Major ATP-Generating Process
Oxidative phosphorylation, encompassing both the electron transport chain and chemiosmosis, represents the major ATP-generating process in mitochondria. This process efficiently harnesses the energy stored in NADH and FADH2 to produce a substantial amount of ATP.
Substrate-Level Phosphorylation: Direct Phosphate Transfer
Substrate-level phosphorylation is a less prevalent mechanism of ATP synthesis, involving the direct transfer of a phosphate group from a high-energy substrate molecule to ADP. This process occurs in both glycolysis and the Krebs cycle, contributing to the overall ATP yield.
Photosynthesis: Capturing Light Energy to Synthesize ATP
Photosynthesis is the process by which plants, algae, and certain bacteria convert light energy into chemical energy in the form of glucose. This process also generates ATP, which is essential for the subsequent steps of carbohydrate synthesis.
Light-Dependent Reactions: Converting Light to Chemical Energy
The light-dependent reactions occur in the thylakoid membranes of chloroplasts. Light energy is absorbed by chlorophyll and other pigments, driving the splitting of water molecules and releasing oxygen. This process also generates ATP and NADPH, an electron carrier similar to NADH.
Calvin Cycle: Fixing Carbon Dioxide into Sugar
The Calvin cycle takes place in the stroma of chloroplasts. In this cycle, carbon dioxide from the atmosphere is fixed and converted into glucose using the ATP and NADPH generated during the light-dependent reactions. Thus, ATP and NADPH produced in the light-dependent reaction is the energy source for Carbon Fixation in the Calvin Cycle.
The Role of Glucose as a Primary Fuel Source
Glucose serves as a primary fuel source for ATP production in most organisms. Its high energy content and relative abundance make it an ideal substrate for both cellular respiration and photosynthesis. Glucose provides the carbon backbone and electrons needed for ATP synthesis.
The Involvement of NAD+/NADH and FAD/FADH2
NAD+/NADH and FAD/FADH2 are crucial coenzymes that participate in redox reactions during cellular respiration. NAD+ and FAD accept electrons during glycolysis and the Krebs cycle, becoming NADH and FADH2, respectively. These reduced coenzymes then donate their electrons to the electron transport chain, driving the production of ATP through oxidative phosphorylation. NADH and FADH2 effectively act as energy shuttles, transferring electrons from glucose oxidation to the ATP-generating machinery. Their regenerative properties are critical for the continuous cycling of cellular respiration.
ATP in Cellular Processes: Fueling Life's Activities
[ATP Synthesis: Cellular Respiration and Photosynthesis - The Energy Generation Pathways Having explored the mechanism by which ATP releases energy, it is imperative to investigate the reverse process: ATP synthesis. This section elucidates the two dominant pathways responsible for generating ATP in biological systems: cellular respiration and photo...]
ATP's role transcends merely serving as a short-term energy storage unit; it is a dynamic participant in a myriad of cellular functions, underpinning the very essence of life. From enabling macroscopic movements like muscle contraction to orchestrating microscopic events such as active transport across cellular membranes and mediating complex signaling cascades, ATP's versatility is unparalleled. Understanding its precise involvement in these processes is paramount to appreciating cellular physiology.
Muscle Contraction: The Actin-Myosin Dance Powered by ATP
The coordinated contraction of muscle tissue, responsible for locomotion, respiration, and myriad other physiological functions, is critically dependent on ATP. This process is primarily driven by the interaction between two key proteins: actin and myosin. Myosin, acting as a molecular motor, binds to actin filaments to generate force and cause muscle shortening.
ATP’s crucial role lies in both enabling the myosin head to bind to actin and subsequently causing its detachment, thus allowing the cycle of attachment, force generation, and detachment to continue. In the absence of ATP, myosin remains tightly bound to actin, resulting in rigor mortis, a testament to ATP's indispensable function.
The Power Stroke
The hydrolysis of ATP provides the energy for the "power stroke," the conformational change in the myosin head that pulls the actin filament, resulting in muscle contraction. The release of ADP and inorganic phosphate (Pi) following the power stroke further reinforces the binding of myosin to actin, perpetuating the contractile cycle.
Active Transport: Breaching the Concentration Gradient
Cellular membranes, composed of a lipid bilayer, inherently restrict the free passage of many essential molecules and ions. Active transport mechanisms, which require energy input, are therefore critical for maintaining appropriate intracellular concentrations.
ATP directly fuels these transport processes, often through the action of specialized transmembrane proteins known as ATPases. These proteins harness the energy released from ATP hydrolysis to pump ions or molecules against their concentration gradients.
Sodium-Potassium Pump: An Illustrative Example
A quintessential example of ATP-dependent active transport is the sodium-potassium (Na+/K+) pump. This pump maintains the electrochemical gradient across the plasma membrane by exporting three sodium ions from the cell and importing two potassium ions, both against their respective concentration gradients. The cycle relies on the phosphorylation of the pump by ATP, which induces conformational changes essential for ion translocation.
Signal Transduction: ATP as a Phosphorylation Agent
Cellular communication is a complex process involving intricate signaling pathways. ATP plays a pivotal role in these pathways, primarily as a substrate for kinases, enzymes that catalyze the transfer of a phosphate group from ATP to a target protein.
This phosphorylation event can alter the target protein's activity, localization, or interaction with other proteins, thereby propagating the signal downstream. Kinases are central to various signaling cascades, including those involved in cell growth, differentiation, and apoptosis.
The Ubiquitous Role of Protein Kinases
The reversible nature of phosphorylation, mediated by phosphatases that remove phosphate groups, allows for dynamic regulation of cellular processes. The interplay between kinases and phosphatases constitutes a fundamental mechanism for controlling protein function and cellular behavior. Dysregulation of kinase activity is frequently implicated in various diseases, including cancer, underscoring the critical role of ATP-dependent phosphorylation in maintaining cellular homeostasis.
Phosphorylation and Dephosphorylation: A Dynamic Duo
The balance between phosphorylation and dephosphorylation serves as a critical regulatory mechanism within cells. Phosphorylation, the addition of a phosphate group to a protein (typically on serine, threonine, or tyrosine residues), is primarily mediated by kinases, as mentioned above.
Dephosphorylation, the removal of this phosphate group, is carried out by phosphatases. These two opposing processes allow cells to rapidly and reversibly control protein activity, localization, and interactions. The dynamic interplay between phosphorylation and dephosphorylation is essential for maintaining cellular homeostasis and responding to external stimuli.
ATP: The Metabolic Hub
ATP is not merely a fuel; it is the central currency in the energetic economy of the cell. It connects catabolic pathways (breaking down molecules to release energy) with anabolic pathways (building complex molecules). Catabolic processes such as glycolysis and oxidative phosphorylation generate ATP, while anabolic processes, like protein synthesis and DNA replication, consume ATP.
The ubiquitous involvement of ATP in diverse metabolic reactions underscores its essential role in maintaining the cellular machinery and driving the processes necessary for life. Any significant disruption in ATP homeostasis can have severe consequences, leading to cellular dysfunction and even death. Therefore, the tight regulation of ATP production and consumption is crucial for cellular survival.
ATP Regulation and Dynamics: Maintaining Cellular Equilibrium
Having illustrated the diverse roles ATP plays in fueling cellular activities, an equally critical aspect is how cells regulate ATP levels to ensure efficient function and prevent energy imbalances. This section delves into the intricate mechanisms governing ATP homeostasis, emphasizing the roles of enzyme kinetics, allosteric regulation, and the critical ATP/ADP ratio.
Cellular ATP Homeostasis: A Delicate Balance
Maintaining a stable ATP concentration is paramount for cellular survival. Fluctuations in ATP levels can disrupt cellular processes, leading to metabolic dysfunction and even cell death.
The cellular ATP concentration is not static. It is in dynamic equilibrium, constantly being synthesized and consumed.
This balance is tightly controlled through various regulatory mechanisms, ensuring that energy supply meets energy demand.
Enzyme Kinetics: Fine-Tuning ATP-Dependent Reactions
Enzyme kinetics plays a pivotal role in regulating ATP-dependent reactions. The activity of many enzymes involved in both ATP synthesis and ATP utilization is highly sensitive to ATP concentration.
For example, enzymes involved in glycolysis, the initial stage of glucose metabolism, are often allosterically regulated by ATP.
High ATP levels can inhibit these enzymes, slowing down glycolysis and reducing ATP production, preventing overproduction when energy is abundant.
Conversely, low ATP levels can stimulate these enzymes, increasing ATP production when energy is scarce.
Allosteric Regulation: A Key Regulatory Mechanism
Allosteric regulation is a crucial mechanism for fine-tuning enzyme activity based on cellular energy status. Many enzymes involved in ATP-utilizing and ATP-generating pathways possess allosteric sites that bind ATP, ADP, or AMP.
These binding events can alter the enzyme's conformation, affecting its affinity for substrates and its overall catalytic activity.
For instance, phosphofructokinase-1 (PFK-1), a key enzyme in glycolysis, is inhibited by ATP and citrate, indicating high energy availability, and activated by AMP, signaling energy depletion.
This intricate allosteric control ensures that ATP production is precisely matched to cellular energy needs.
The Critical ATP/ADP Ratio: A Metabolic Indicator
The ratio of ATP to ADP (and AMP) serves as a key indicator of the cell's energy state. A high ATP/ADP ratio signals an abundance of energy, while a low ratio indicates energy depletion.
Cells maintain a tightly regulated ATP/ADP ratio to ensure optimal metabolic function.
This ratio influences the activity of numerous enzymes and regulatory proteins.
Deviations from the optimal ATP/ADP ratio can trigger compensatory mechanisms, such as activating catabolic pathways to generate more ATP or inhibiting anabolic pathways to conserve energy.
Implications of Dysregulated ATP Homeostasis
Disruptions in ATP regulation can have severe consequences for cellular function and organismal health.
Conditions such as ischemia (reduced blood flow) and hypoxia (oxygen deprivation) can impair ATP synthesis, leading to a rapid depletion of ATP levels.
This energy crisis can trigger cellular damage and ultimately cell death.
Furthermore, dysregulation of ATP metabolism has been implicated in various diseases, including cancer, neurodegenerative disorders, and metabolic syndromes.
Understanding the intricate mechanisms governing ATP regulation is, therefore, crucial for developing therapeutic strategies to combat these diseases.
Techniques for Studying ATP: Unveiling Cellular Energy Dynamics
Having illustrated the diverse roles ATP plays in fueling cellular activities, an equally critical aspect is how cells regulate ATP levels to ensure efficient function and prevent energy imbalances. This section delves into the intricate mechanisms governing ATP homeostasis, emphasizing the methodologies employed to study ATP, with a particular focus on ATP biosensors.
ATP Biosensors: Real-Time Monitoring of Cellular Energy
The study of ATP dynamics within living cells has been revolutionized by the advent of ATP biosensors. These sophisticated tools allow researchers to monitor ATP concentrations in real-time, providing unprecedented insights into cellular energy metabolism and its regulation.
Traditional methods for measuring ATP, such as bioluminescence assays, often require cell lysis. This destructive process provides only a snapshot of ATP levels at a specific time point. In contrast, biosensors offer a non-invasive approach, enabling continuous monitoring of ATP fluctuations within intact cells.
Types of ATP Biosensors
Several types of ATP biosensors have been developed, each with its own advantages and limitations. These include:
Fluorescent Protein-Based Biosensors
These sensors rely on genetically encoded fluorescent proteins that change their fluorescence properties in response to ATP binding. A common design involves fusing an ATP-binding protein to a fluorescent protein, such as yellow fluorescent protein (YFP) or cyan fluorescent protein (CFP).
When ATP binds, the conformational change in the ATP-binding protein alters the fluorescence resonance energy transfer (FRET) between CFP and YFP, resulting in a detectable change in the fluorescence signal. These biosensors offer high sensitivity and can be targeted to specific cellular compartments.
Bioluminescent Biosensors
Bioluminescent biosensors utilize the enzyme luciferase, which catalyzes a light-emitting reaction in the presence of ATP and luciferin. By engineering luciferase to be more sensitive to ATP or by combining it with other ATP-binding proteins, researchers can create biosensors that report on ATP levels through bioluminescence.
Electrochemical Biosensors
Electrochemical biosensors use electrodes modified with ATP-binding molecules to detect changes in electrical current or potential upon ATP binding. These sensors offer the potential for high sensitivity and miniaturization but are less commonly used for intracellular ATP measurements.
Applications of ATP Biosensors
ATP biosensors have found widespread applications in various fields of biology and medicine. They have been used to study:
-
Metabolic regulation: Understanding how cells respond to changes in nutrient availability or energy demand.
-
Mitochondrial function: Monitoring ATP production within mitochondria and identifying mitochondrial dysfunction in disease.
-
Cellular signaling: Investigating the role of ATP in signaling pathways and cellular communication.
-
Drug discovery: Screening for compounds that affect ATP metabolism or cellular energy balance.
Challenges and Future Directions
Despite their power, ATP biosensors also face several challenges. One major challenge is the potential for biosensors to perturb cellular ATP levels or interfere with normal cellular function. Careful design and validation are necessary to minimize these effects.
Another challenge is the limited availability of biosensors with optimal sensitivity and dynamic range for all cellular contexts. Future research efforts are focused on developing new and improved ATP biosensors with enhanced properties, as well as expanding their application to new areas of study.
The continued development and application of ATP biosensors promise to further illuminate the intricate dynamics of cellular energy metabolism and provide new insights into human health and disease.
Future Directions and Current Research: Exploring the Frontiers of ATP Biology
Having introduced the techniques used to study ATP and unveil its cellular energy dynamics, the next logical step is to explore the ongoing research that is pushing the boundaries of our understanding of this vital molecule. Current investigations are not only refining our knowledge of ATP metabolism and regulation but also uncovering novel roles for ATP in previously unappreciated cellular processes. This section highlights some of these exciting avenues of research, showcasing the innovative work of scientists at the forefront of ATP biology.
Unraveling ATP Metabolism in Cancer
Cancer cells exhibit altered metabolic profiles to support their rapid proliferation and survival. A key area of intense research focuses on understanding how cancer cells manipulate ATP metabolism to meet their energy demands.
Researchers are investigating the enzymes involved in glycolysis, the Krebs cycle, and oxidative phosphorylation in cancer cells, seeking to identify potential therapeutic targets.
Specific isozymes of these enzymes, which are uniquely expressed or upregulated in cancer, are of particular interest.
Furthermore, the role of mitochondrial dynamics and function in cancer cell ATP production is being actively explored.
The Warburg Effect Revisited
The Warburg effect, the observation that cancer cells preferentially utilize glycolysis even in the presence of oxygen, is being re-examined with advanced tools and perspectives.
Researchers are now investigating the precise mechanisms that drive this metabolic shift and its implications for cancer progression and treatment resistance.
Understanding the nuances of ATP generation pathways in different cancer types could pave the way for personalized cancer therapies targeting their specific metabolic vulnerabilities.
ATP in Neurotransmission and Neurological Disorders
ATP is not merely an energy source; it also functions as a neurotransmitter in both the central and peripheral nervous systems. This non-canonical role of ATP has garnered significant attention in recent years.
Researchers are investigating the release, signaling, and breakdown of ATP in neurons and glial cells.
Dysregulation of ATP signaling has been implicated in various neurological disorders, including pain, epilepsy, and neurodegenerative diseases.
Purinergic Receptors as Therapeutic Targets
Purinergic receptors, which bind ATP and other nucleotides, are being explored as potential therapeutic targets for neurological conditions.
Specific subtypes of purinergic receptors are expressed in distinct brain regions and cell types, allowing for targeted interventions.
Modulating ATP signaling through purinergic receptors holds promise for developing novel treatments for chronic pain, neuroinflammation, and other neurological disorders.
The Role of ATP in Inflammatory Responses
ATP is released from damaged cells and can act as a "danger signal," triggering inflammatory responses through the activation of inflammasomes.
Inflammasomes are multiprotein complexes that initiate the maturation and release of pro-inflammatory cytokines, such as IL-1β and IL-18.
Researchers are investigating the role of ATP-dependent inflammasome activation in various inflammatory diseases, including arthritis, atherosclerosis, and inflammatory bowel disease.
ATP as a Mediator of Sterile Inflammation
Sterile inflammation, which occurs in the absence of infection, can be driven by the release of intracellular molecules, including ATP, from damaged cells.
Targeting ATP release or its downstream signaling pathways may offer a therapeutic strategy for controlling sterile inflammation in conditions such as trauma and ischemia-reperfusion injury.
ATP and Aging
The intricate relationship between ATP metabolism and aging is an emerging area of investigation.
Age-related decline in mitochondrial function and ATP production contributes to cellular dysfunction and the development of age-related diseases.
Enhancing mitochondrial function and ATP synthesis may be a promising strategy for promoting healthy aging and extending lifespan.
NAD+ Augmentation and ATP Production
Nicotinamide adenine dinucleotide (NAD+) is a crucial coenzyme involved in ATP production, DNA repair, and other essential cellular processes.
NAD+ levels decline with age, contributing to mitochondrial dysfunction and reduced ATP synthesis.
Strategies to boost NAD+ levels, such as supplementation with NAD+ precursors, are being investigated for their potential to improve mitochondrial function and promote healthy aging.
Video: ATP vs ADP: Energy Currency (Adenosine Triphosphate)
ATP vs ADP: Energy Currency FAQs
What is the primary difference between ATP and ADP regarding energy?
ATP (adenosine triphosphate) holds more chemical energy than ADP (adenosine diphosphate). ATP has three phosphate groups, while ADP has only two. The breaking of the bond between the last two phosphate groups in ATP releases energy, converting adenosine triphosphate v adp.
How does ATP become ADP, and why is this important?
ATP becomes ADP when a phosphate group is removed, releasing energy for cellular work. This process, called hydrolysis, is crucial because it provides the energy for various cellular functions, effectively shifting adenosine triphosphate v adp.
What role does ADP play in regenerating ATP?
ADP is essential for regenerating ATP. Through cellular respiration or photosynthesis, energy is used to reattach a phosphate group to ADP, creating ATP, a key part of adenosine triphosphate v adp cycling.
Where does the phosphate group come from when ADP is converted to ATP?
The phosphate group needed to convert ADP back into ATP comes from other molecules within the cell, often derived from the breakdown of nutrients. This process requires energy input and effectively replenishes the cell's energy currency of adenosine triphosphate v adp.
So, next time you're crushing it at the gym, or even just thinking really hard, remember all the amazing work going on at the molecular level with adenosine triphosphate vs ADP. It's pretty wild to think that such tiny molecules are powering everything we do!