Is Air Resistance a Contact Force? Science
Air resistance, a ubiquitous force, poses a fascinating question within the realm of physics: is air resistance a contact force? Understanding this phenomenon requires delving into the microscopic interactions between air molecules and the surface of a moving object. Aerodynamics, a branch of fluid dynamics, investigates how air flows around objects, influencing the magnitude of air resistance. Isaac Newton's laws of motion provide the foundational principles for analyzing forces, including the determination of whether interactions, such as those in air resistance, qualify as contact forces. The National Aeronautics and Space Administration (NASA) conducts extensive research on air resistance to optimize the design of aircraft and spacecraft, further highlighting the practical implications of determining if air resistance is a contact force.
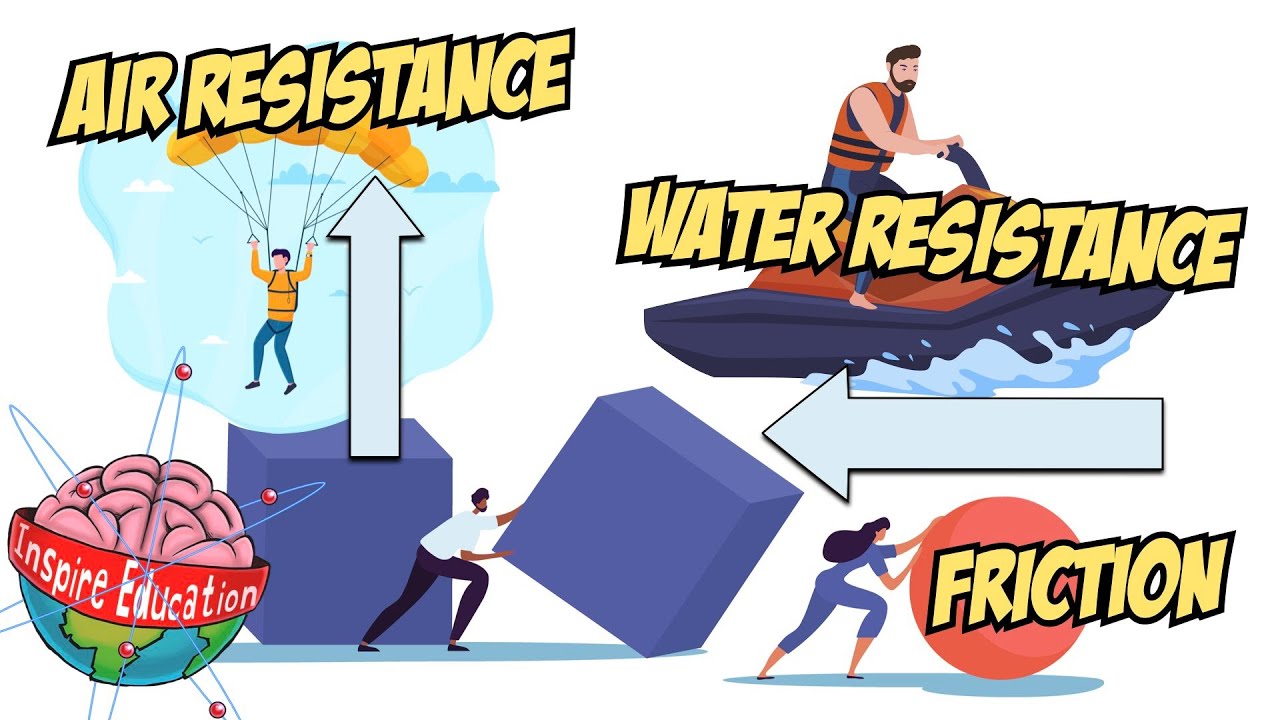
Image taken from the YouTube channel Inspire Education , from the video titled Forces I Friction, Air Resistance and Water Resistance .
Unveiling the Invisible Force of Air Resistance
Air resistance, a force often overlooked, is fundamental to understanding the motion of objects through the atmosphere.
It's the invisible hand that slows a skydiver's descent, shapes the design of a high-speed train, and determines the trajectory of a baseball.
This opposing force, seemingly simple, is governed by complex interactions between an object and the air it traverses. Its understanding unlocks countless possibilities across diverse fields.
The Pervasive Influence of Air Resistance
From the intricacies of engineering to the nuances of physics and the thrill of sports, air resistance plays a pivotal role.
Engineers meticulously consider it when designing vehicles, striving to minimize drag and maximize fuel efficiency.
Physicists study its effects to refine models of motion and understand the behavior of fluids.
Athletes and designers of sporting equipment are constantly seeking ways to harness or minimize air resistance to gain a competitive edge.
Charting the Course: A Roadmap of Air Resistance
To fully grasp the significance of air resistance, we must delve into its underlying principles.
We will explore its relationship with velocity and surface area.
We will also examine the crucial distinction between laminar and turbulent flow.
Finally, we'll consider practical methods for measuring its magnitude. We will explore how this understanding impacts fields as varied as vehicle design and competitive sports.
The Foundation: Forces, Contact, and Newton's Laws
Air resistance, a force often overlooked, is fundamental to understanding the motion of objects through the atmosphere. It's the invisible hand that slows a skydiver's descent, shapes the design of a high-speed train, and determines the trajectory of a baseball. This opposing force, seemingly simple, rests upon the bedrock of fundamental physics principles, including the concept of force itself and the laws governing motion.
Understanding Force: The Agent of Change
At its core, a force is an interaction that, when unopposed, will change the motion of an object. More precisely, force is defined as a push or pull that can cause an object to accelerate, decelerate, or change direction. It's the agent responsible for altering an object's state of motion.
Without a net force acting upon it, an object will either remain at rest or continue moving at a constant velocity, as dictated by Newton's First Law.
Contact Forces: Distinguishing the Tangible
To better understand air resistance, it's crucial to distinguish it from other types of forces, particularly contact forces.
Contact forces, as the name suggests, arise from direct physical contact between objects. Examples include:
- Friction: The force resisting the relative motion of solid surfaces, fluid layers, or elements sliding against each other.
- Tension: The force transmitted through a string, rope, cable, or wire when it is pulled tight by forces acting from opposite ends.
- Normal Force: The force exerted by a surface that supports the weight of an object.
Air resistance, while also a resistive force, differs from these in that it arises from the interaction between an object and the fluid (air) through which it moves. It's a distributed force acting over the object's surface, rather than a concentrated force at a point of contact.
Newton's Laws of Motion: The Framework for Understanding Air Resistance
Newton's Laws of Motion provide the essential framework for understanding how air resistance affects the motion of objects. While all three laws are relevant, Newton's Second Law is particularly insightful:
Newton's Second Law
This law states that the acceleration of an object is directly proportional to the net force acting on it and inversely proportional to its mass.
Mathematically, this is expressed as:
F = ma
Where:
- F represents the net force acting on the object.
- m is the mass of the object.
- a is the acceleration of the object.
Air resistance contributes to the 'F' (net force) term in this equation. As an object moves through the air, the force of air resistance opposes its motion, effectively reducing the net force and, consequently, the acceleration.
For example, consider a ball dropped from a height. Initially, the only force acting on it is gravity, causing it to accelerate downwards. As the ball gains speed, air resistance increases, opposing the gravitational force. The net force on the ball decreases, and its acceleration diminishes. Eventually, air resistance may equal the gravitational force, resulting in zero net force and zero acceleration. The ball then falls at a constant velocity, known as terminal velocity.
Therefore, understanding the principles of force, contact forces, and Newton's Laws – especially the Second Law – is paramount to grasping the dynamics of air resistance and its profound impact on the motion of objects in our everyday world.
Air Resistance Defined: Drag, Velocity, and Surface Area
Air resistance, a force often overlooked, is fundamental to understanding the motion of objects through the atmosphere. It's the invisible hand that slows a skydiver's descent, shapes the design of a high-speed train, and determines the trajectory of a baseball. This opposing force, seemingly simple, is actually a complex interaction between an object and the air it moves through. To truly grasp its effects, we must delve into its definition and the key factors influencing its magnitude: drag, velocity, and surface area.
Drag: The Cumulative Resistance
The terms "air resistance" and "drag" are often used interchangeably, and for good reason. Drag represents the cumulative resistive forces exerted by a fluid – in this case, air – on a moving object. It's not a single point of contact, but rather the sum of countless tiny interactions between air molecules and the object's surface.
Think of it like this: each air molecule that collides with the object exerts a small force. The collective effect of all these collisions, happening simultaneously across the entire surface, is what we perceive as drag.
This force always acts in the direction opposite to the object's motion, constantly working to slow it down. Understanding drag as a cumulative effect is crucial for accurately predicting and controlling motion in aerodynamic environments.
Velocity: The Quadratic Relationship
The relationship between an object's velocity and the magnitude of air resistance is not linear; it's quadratic. This means that as the velocity increases, the air resistance increases exponentially, not proportionally.
Specifically, air resistance is proportional to the square of the velocity. So, if you double the speed of an object, the air resistance force quadruples. This has profound implications for high-speed motion.
Why this quadratic relationship? At higher speeds, the object collides with more air molecules per unit time, and each collision is more forceful. This intensified interaction leads to a much greater resistive force.
This principle is critical in fields like automotive and aerospace engineering, where even small increases in speed can dramatically increase fuel consumption due to the amplified effect of drag.
Surface Area: The Exposed Face
The surface area of an object exposed to the airflow also plays a crucial role in determining the magnitude of air resistance. A larger surface area means there's more space for air molecules to collide with, leading to a greater overall drag force.
Imagine holding a flat piece of cardboard out of a car window. If you hold it perpendicular to the wind, the resistance is much greater than if you hold it edge-on. The difference is the amount of surface area interacting with the airflow.
The orientation of the object matters just as much as its size. A streamlined object, despite having a similar overall size, can present a significantly smaller effective surface area to the airflow, thereby reducing drag.
This is why airplanes are designed with long, narrow wings and cars are often given teardrop shapes; to minimize the frontal surface area and reduce the impact of air resistance. Minimizing the effective surface area becomes a key design objective to increase efficiency and maximize performance.
Pressure's Role: Understanding Form Drag
Air resistance, a force often overlooked, is fundamental to understanding the motion of objects through the atmosphere. It's the invisible hand that slows a skydiver's descent, shapes the design of a high-speed train, and determines the trajectory of a baseball. This opposing force, seemingly simple, is in reality a complex interplay of factors, with pressure playing a pivotal role in a phenomenon known as form drag.
Form drag, also called pressure drag, emerges from the pressure differences created as an object moves through the air. Unlike skin friction drag, which arises from the air's viscosity interacting with the object's surface, form drag is dictated by the object's shape and its effect on the surrounding airflow.
The Mechanics of Form Drag
Imagine air flowing around an object. At the front, the air compresses, creating a region of high pressure. As the air flows around the object's sides, it must accelerate. If the object is streamlined, the airflow remains smooth, and the pressure gradually recovers towards the back.
However, if the object is blunt, the air separates from the surface, creating a turbulent wake behind it. This wake is characterized by low pressure. The pressure difference between the high-pressure front and the low-pressure wake results in a net force opposing the object's motion – form drag.
Pressure Differentials and Air Resistance
The magnitude of form drag is directly proportional to the pressure difference between the front and rear of the object. A large pressure difference signifies a substantial force impeding movement. This is why the shape of an object is so crucial.
A streamlined shape minimizes the pressure difference by allowing the air to flow smoothly and maintain relatively high pressure at the rear. A blunt shape, conversely, maximizes the pressure difference by creating a large, low-pressure wake.
Shape Matters: Examples of Form Drag
Consider these examples to illustrate the impact of shape:
- Aerofoil vs. Flat Plate: An aerofoil (like an airplane wing) is designed to minimize form drag by smoothly guiding airflow and promoting pressure recovery. A flat plate perpendicular to the airflow, on the other hand, experiences significant form drag due to the large pressure difference it creates.
- Car Design: Early automobiles were boxy and experienced substantial form drag, limiting their speed and fuel efficiency. Modern cars are designed with smoother, more rounded shapes to reduce form drag and improve performance.
- Skydiver's Position: A skydiver increases their drag by adopting a flat, spread-eagle position, maximizing form drag and slowing their descent. Conversely, a streamlined, head-down position minimizes form drag, allowing them to reach higher speeds.
These examples demonstrate that an object's shape is not merely aesthetic; it is a critical determinant of the amount of air resistance it experiences. Understanding form drag is essential for designing efficient vehicles, optimizing athletic performance, and predicting the motion of objects through the air.
Fluid Dynamics and Aerodynamics: The Science Behind Airflow
Air resistance, a force often overlooked, is fundamental to understanding the motion of objects through the atmosphere. It's the invisible hand that slows a skydiver's descent, shapes the design of a high-speed train, and determines the trajectory of a baseball. This opposing force, seemingly simple, is in reality governed by complex principles rooted in fluid dynamics and its specialized offspring, aerodynamics.
To truly grasp the nature of air resistance, we must first acknowledge the fundamental nature of air itself: it is a fluid.
Air as a Fluid: The Realm of Fluid Dynamics
Fluid dynamics is the branch of physics that deals with the motion of liquids and gases. Its principles provide the essential framework for understanding how air interacts with objects moving through it.
Key concepts, such as viscosity (a fluid's resistance to flow), pressure, and flow velocity, all play a crucial role. These properties determine how air flows around an object, creating regions of high and low pressure that ultimately contribute to the overall force of air resistance.
Considering air as a fluid is paramount to understanding the forces it exerts on objects moving within it. Air's properties influence everything from lift generation on an airplane wing to the drag experienced by a cyclist.
Aerodynamics: Focusing on Air
While fluid dynamics provides the overarching theory, aerodynamics narrows its focus specifically to the study of air and its interaction with moving objects.
Aerodynamics delves into the intricate details of airflow, analyzing phenomena like boundary layers, turbulence, and pressure gradients.
It is the bedrock of aircraft design, automotive engineering, and even sports equipment development, providing insights into how to minimize drag and maximize efficiency.
The Significance of Streamlining
A central theme in aerodynamics is the concept of streamlining. Streamlined shapes are designed to minimize turbulence and promote smooth, laminar airflow.
This reduces pressure differences and lowers the overall drag force, enabling objects to move more efficiently through the air.
Conversely, blunt or irregular shapes tend to create significant turbulence, leading to increased drag and reduced performance.
The Role of Simulation and Experimentation
Aerodynamic principles are not merely theoretical constructs. They are constantly refined and validated through sophisticated simulations and meticulous experimentation.
Computational Fluid Dynamics (CFD) allows engineers to model airflow around complex shapes, predicting drag and lift forces with remarkable accuracy. Wind tunnels provide a controlled environment for real-world testing, allowing for the measurement of these forces and the optimization of designs.
The interplay between theoretical understanding, computational modeling, and experimental validation is the cornerstone of modern aerodynamics, driving innovation in diverse fields and enhancing our ability to move through the air with greater efficiency and control.
Laminar vs. Turbulent Flow: A Tale of Two Airflows
Air resistance, a force often overlooked, is fundamental to understanding the motion of objects through the atmosphere. It's the invisible hand that slows a skydiver's descent, shapes the design of a high-speed train, and determines the trajectory of a baseball. This opposing force, seemingly simple, is governed by complex interactions between the moving object and the air surrounding it. One of the most crucial aspects of these interactions is the nature of airflow itself – whether it's smooth and ordered (laminar) or chaotic and swirling (turbulent). Understanding the difference is key to minimizing drag and maximizing efficiency.
The Essence of Laminar Flow
Laminar flow, derived from the Latin word "lamina" meaning layer, describes a state where air moves in smooth, parallel layers. Imagine a deck of cards perfectly aligned; each card represents a layer of air smoothly sliding over the one beneath it. In this regime, the air molecules follow predictable paths, minimizing friction and energy loss. This smooth, layered movement translates directly to lower drag, as the object encounters less resistance from the air it passes through.
Consider the sleek, tapered design of a glider's wing. This shape is meticulously crafted to encourage laminar flow over its surface. The smooth airflow reduces drag, allowing the glider to soar effortlessly through the air, maximizing its distance and time aloft.
The Chaotic Nature of Turbulent Flow
In stark contrast to laminar flow, turbulent flow is characterized by chaotic, swirling air movement. Instead of smooth layers, the air becomes a jumbled mix of eddies and vortices, creating a complex and unpredictable flow field. This irregular motion significantly increases friction and energy dissipation, leading to higher drag. Turbulent flow arises when the air's velocity exceeds a critical threshold, or when the object's shape disrupts the smooth flow.
Imagine a rock in a stream. The water flows smoothly around the front of the rock, but behind it, the flow breaks down into swirling eddies and chaotic currents. This is a visual representation of turbulent flow.
Visualizing the Difference
The contrast between laminar and turbulent flow is often best understood visually.
Depicting Laminar Flow
Laminar flow can be imagined as neatly organized streamlines of airflow. Each streamline represents the path of a particle of air, and in laminar flow, these streamlines are parallel and uniform. Dye injected into a laminar flow will form a smooth, unbroken line.
Depicting Turbulent Flow
Turbulent flow, on the other hand, is characterized by chaotic, interwoven streamlines. Dye injected into a turbulent flow will quickly disperse and mix, revealing the swirling eddies and vortices that define this type of flow.
The Impact on Drag
The difference between laminar and turbulent flow has a profound impact on the overall drag experienced by an object. Laminar flow minimizes drag, while turbulent flow significantly increases it. This is because turbulent flow creates a larger wake behind the object, a region of low pressure that pulls backward on the object.
Boundary Layer Transition: The Shift from Order to Chaos
The transition from laminar to turbulent flow often occurs within a boundary layer, a thin layer of air directly adjacent to the object's surface. At the leading edge of the object, the flow is typically laminar. However, as the flow progresses along the surface, it can become unstable and transition to turbulence. The location of this transition point is crucial in determining the overall drag. Delaying the transition to turbulent flow is a key strategy in aerodynamic design. Strategies such as using a smooth surface or implementing boundary layer suction delay this transition.
The Role of Shape
The shape of an object plays a critical role in determining whether the airflow around it will be laminar or turbulent. Streamlined shapes, like those found in aircraft wings and high-performance cars, are designed to promote laminar flow and minimize drag. These shapes gradually deflect the air, allowing it to flow smoothly around the object's surface.
Blunt shapes, on the other hand, tend to disrupt the airflow and promote turbulence. The sharp edges of a blunt object cause the air to separate from the surface, creating a large turbulent wake behind the object. This wake increases drag and reduces efficiency.
Factors Influencing Air Resistance: Shape and Density
Air resistance, a force often overlooked, is fundamental to understanding the motion of objects through the atmosphere. It's the invisible hand that slows a skydiver's descent, shapes the design of a high-speed train, and determines the trajectory of a baseball. This opposing force, seemingly simple on the surface, is governed by a complex interplay of factors, most notably an object's shape and the density of the air it traverses.
These elements exert a profound influence on the magnitude of air resistance, dictating the efficiency of movement and impacting everything from fuel consumption to athletic performance. Understanding these factors is crucial for anyone seeking to optimize motion within a fluid environment.
The Role of Shape: Streamlining and Airflow
An object's shape is arguably the most critical determinant of air resistance. The principle at play here is the manner in which air flows around the object. Streamlined shapes, characterized by smooth, gradually curving surfaces, are designed to minimize disruption to the airflow.
Such designs encourage laminar flow, where air particles move in smooth, parallel layers with minimal mixing or turbulence. This orderly flow significantly reduces drag, as the air can smoothly separate from the object's surface without creating large pressure differences.
Think of the cross-section of an airplane wing or the sleek body of a racing car; these forms are carefully sculpted to encourage laminar flow and minimize energy loss due to air resistance. The result is greater efficiency and higher speeds.
Blunt Shapes and Turbulent Wake
In stark contrast, blunt or irregular shapes present a significant obstacle to the smooth flow of air. When air encounters a blunt object, it is forced to abruptly change direction, leading to separation from the surface and the formation of a turbulent wake.
This wake is characterized by chaotic, swirling air currents and areas of low pressure behind the object. The pressure difference between the front and rear of the object creates a strong drag force, significantly increasing air resistance.
Consider a parachute or a flat plate moving through the air; these shapes generate substantial turbulence, maximizing drag and quickly decelerating the object. This effect is exploited in applications where rapid deceleration is desired, but it is a major hindrance in scenarios prioritizing speed and efficiency.
Air Density: A Matter of Altitude and Temperature
While shape dictates how air flows around an object, air density determines the mass of air that must be displaced. Air density, in turn, is heavily influenced by both altitude and temperature.
At higher altitudes, the air is less dense, meaning there are fewer air molecules per unit volume. Consequently, an object moving through less dense air encounters less resistance. This is why airplanes often fly at high altitudes, where the reduced air resistance allows for greater fuel efficiency.
Temperature also plays a role; warmer air is less dense than colder air. As air heats up, its molecules move faster and spread out, decreasing the number of molecules within a given volume. This means that an object moving through warmer air will experience slightly less air resistance than it would in colder air at the same altitude.
The combined effect of altitude and temperature can significantly impact air resistance, especially in applications where precision and performance are paramount. Therefore, engineers and athletes often consider these environmental factors when designing equipment or strategizing for optimal performance.
Measuring Air Resistance: Wind Tunnels, Anemometers, and Force Sensors
Air resistance, a force often overlooked, is fundamental to understanding the motion of objects through the atmosphere. It's the invisible hand that slows a skydiver's descent, shapes the design of a high-speed train, and determines the trajectory of a baseball. This opposing force, seemingly simple, requires sophisticated measurement techniques to fully comprehend its effects. Understanding and quantifying air resistance requires a diverse set of tools, ranging from controlled laboratory environments to real-world observational devices. These methods allow scientists and engineers to accurately assess and predict the aerodynamic performance of various objects.
The Wind Tunnel: A Controlled Aerodynamic Laboratory
The wind tunnel stands as a cornerstone in aerodynamic research. It provides a controlled environment where objects can be subjected to precise airflow conditions, replicating various real-world scenarios. Within a wind tunnel, powerful fans generate a consistent stream of air, the speed of which can be carefully regulated.
Objects, ranging from scale models of aircraft to full-sized vehicles, are placed within the tunnel's test section. Sophisticated instrumentation then measures the forces exerted by the airflow.
How Wind Tunnels Work
At its core, a wind tunnel simulates the relative motion between an object and the air surrounding it. By keeping the object stationary and moving the air, researchers can isolate and study the aerodynamic forces at play. This setup allows for meticulous data collection without the complexities of real-world movement.
Wind Tunnel Instrumentation
Wind tunnels are equipped with a variety of sensors to quantify different aspects of airflow and its interaction with the test object. Pressure sensors measure the distribution of pressure across the object's surface, offering insights into areas of high and low pressure. Force balances precisely measure the overall lift, drag, and side forces acting on the object. These measurements are crucial for optimizing designs and predicting performance.
Anemometers: Measuring Air Speed in the Real World
While wind tunnels offer controlled environments, understanding air resistance also requires measurements in real-world conditions. This is where anemometers come into play. Anemometers are devices designed to measure wind speed, providing crucial data for various applications, from weather forecasting to aviation safety.
Types of Anemometers
Several types of anemometers exist, each with its own strengths and weaknesses. Cup anemometers, with their rotating cups, are widely used for their simplicity and reliability. Hot-wire anemometers, on the other hand, use a heated wire to measure wind speed based on the rate of heat loss. Ultrasonic anemometers use sound waves to determine wind speed and direction with high precision.
Applications of Anemometers
The data collected by anemometers is invaluable across numerous fields. Meteorologists rely on them to track wind patterns and predict weather conditions. Engineers use them to assess the wind loading on structures like bridges and buildings. Pilots depend on accurate wind speed information for safe takeoff and landing.
Force Sensors (Load Cells): Quantifying Air Resistance Directly
While wind tunnels and anemometers provide valuable information about airflow, force sensors, also known as load cells, allow for direct measurement of the forces acting on an object due to air resistance. These sensors are designed to accurately quantify the magnitude of the opposing force.
How Force Sensors Work
Force sensors typically utilize strain gauges, which are small electrical resistors that change their resistance when subjected to strain. When an object experiences air resistance, the force is transmitted to the force sensor, causing a minute deformation. This deformation alters the strain gauge's resistance, which is then translated into a precise force measurement.
Applications of Force Sensors
Force sensors are essential for various applications where accurate measurement of air resistance is crucial. In automotive engineering, they are used to optimize vehicle designs for reduced drag and improved fuel efficiency. In sports science, they help analyze the aerodynamic performance of athletes and equipment, like cyclists and racing suits.
In conclusion, the measurement of air resistance relies on a combination of sophisticated tools and techniques. Wind tunnels provide controlled environments for detailed aerodynamic analysis, while anemometers measure wind speed in real-world conditions. Force sensors, finally, offer a direct means of quantifying the magnitude of air resistance. Together, these tools enable scientists and engineers to understand and manipulate air resistance for a wide range of applications.
Terminal Velocity: The Limit of Falling
Air resistance, a force often overlooked, is fundamental to understanding the motion of objects through the atmosphere. It's the invisible hand that slows a skydiver's descent, shapes the design of a high-speed train, and determines the trajectory of a baseball. This opposing force dramatically influences the maximum speed an object can reach during freefall, a concept known as terminal velocity.
Terminal velocity isn't just an interesting physics phenomenon; it's a critical factor in fields ranging from aerospace engineering to extreme sports. Understanding it provides insights into the delicate balance between gravity and air resistance, revealing the forces that govern our physical world.
Defining Terminal Velocity: Equilibrium in Freefall
Terminal velocity is reached when the force of air resistance acting on a falling object equals the force of gravity pulling it downward. At this point, the net force on the object becomes zero.
This means the object stops accelerating and continues to fall at a constant speed. It's crucial to understand that terminal velocity isn't a fixed value; it varies depending on several factors.
It’s a dynamic equilibrium where the forces of gravity and drag reach a point of balance. This balance dictates the maximum speed achievable during freefall, making it a fundamental concept in physics and engineering.
The Role of Gravity and Air Resistance
Gravity, the force that relentlessly pulls objects towards the Earth, is the driving force behind freefall. However, as an object accelerates downwards, it encounters increasing air resistance.
Air resistance, also known as drag, is a force that opposes motion through the air. The magnitude of this force depends on several factors, including the object's speed, shape, and surface area.
As the object's speed increases, so does the air resistance. Eventually, a point is reached where the upward force of air resistance precisely cancels out the downward force of gravity. This is the point of terminal velocity.
Factors Influencing Terminal Velocity
The terminal velocity of an object isn't a one-size-fits-all value. It's influenced by a complex interplay of several factors. Among the most significant are mass, shape, and air density.
Mass
Mass plays a crucial role in determining terminal velocity. A heavier object experiences a greater force of gravity, requiring a correspondingly larger force of air resistance to reach equilibrium.
Therefore, a more massive object will generally have a higher terminal velocity than a lighter object of the same shape and size. This is because the air resistance needs to be greater to counteract the larger gravitational force.
Shape and Surface Area
The shape and surface area of an object have a profound impact on the amount of air resistance it encounters. A streamlined object, like a teardrop, experiences less air resistance than a flat object, like a parachute.
This is because streamlined shapes promote laminar airflow, reducing drag. Conversely, objects with a large surface area perpendicular to the direction of motion experience greater drag.
This is why parachutes are designed with a large surface area to maximize air resistance and reduce terminal velocity, ensuring a safe landing. The larger the area, the greater the air resistance.
Air Density
Air density, which is affected by factors like altitude and temperature, also influences terminal velocity. At higher altitudes, the air is less dense, resulting in lower air resistance.
Consequently, an object will need to reach a higher speed to generate enough air resistance to balance gravity. This means the terminal velocity of an object will generally be higher at higher altitudes.
Similarly, changes in temperature can affect air density, leading to variations in terminal velocity. Understanding this interplay is crucial in applications like weather forecasting and aviation.
Terminal Velocity in Action: Examples and Implications
The concept of terminal velocity has significant implications in various real-world scenarios:
- Skydiving: Skydivers manipulate their body position to control their surface area and, consequently, their terminal velocity. This allows them to perform aerial maneuvers and safely deploy their parachutes.
- Aerospace Engineering: The design of aircraft and spacecraft must account for air resistance to optimize fuel efficiency and control trajectory.
- Meteorology: Understanding terminal velocity is crucial for predicting the fall speed of raindrops and snowflakes, which is essential for accurate weather forecasting.
In each of these examples, an understanding of terminal velocity allows us to predict and control the motion of objects moving through the air, with practical applications in both engineering and natural sciences.
Real-World Applications: Vehicle Design and Sports
Air resistance, a force often overlooked, is fundamental to understanding the motion of objects through the atmosphere. It's the invisible hand that slows a skydiver's descent, shapes the design of a high-speed train, and determines the trajectory of a baseball. This opposing force dramatically influences the design and strategies across diverse fields, most notably in vehicle engineering and various sports disciplines.
The Quest for Fuel Efficiency: Minimizing Drag in Vehicle Design
In the realm of transportation, minimizing air resistance is paramount for achieving fuel efficiency and reducing emissions. Whether it's a car, a plane, or a train, the battle against drag is a constant engineering challenge. The more aerodynamic a vehicle is, the less energy it expends to overcome air resistance, leading to significant savings and a smaller environmental footprint.
Cars: Streamlining for Efficiency
Automobile manufacturers invest heavily in aerodynamic testing and design. They understand that even subtle changes in a car's shape can have a considerable impact on its fuel consumption.
Sleek profiles, smooth underbodies, and carefully sculpted rear ends are all features designed to reduce turbulence and minimize drag. Wind tunnels are essential tools in this process, allowing engineers to visualize airflow and fine-tune their designs.
Airplanes: Balancing Lift and Drag
Aircraft design presents a unique set of challenges, as engineers must balance the need for lift with the imperative to minimize drag. Airplane wings are shaped to generate lift, but this shape also creates drag.
Optimizing the wing's airfoil and incorporating features like winglets (vertical extensions at the wingtips) can help reduce induced drag, which is created by the generation of lift. Furthermore, streamlining the fuselage and engine nacelles contributes to reduced overall drag.
Trains: High-Speed Aerodynamics
High-speed trains face particularly intense aerodynamic challenges. As speeds increase, air resistance becomes a dominant force, requiring substantial power to overcome.
Designing trains with smooth, streamlined shapes is crucial for reducing drag and improving energy efficiency. Careful attention is paid to the front of the train, where the initial impact with the air occurs.
Gaining the Competitive Edge: Air Resistance in Sports
In sports, even small reductions in air resistance can translate into significant performance gains. Athletes and equipment designers are constantly seeking ways to minimize drag and maximize speed and efficiency.
Cycling: The Art of Aerodynamics
Cycling is perhaps the sport where aerodynamics is most heavily scrutinized. Cyclists spend a significant portion of their energy overcoming air resistance, making aerodynamic optimization crucial for success.
Streamlined helmets, tight-fitting clothing, and aerodynamic bicycle frames are all designed to reduce drag. Even a cyclist's body position on the bike can have a considerable impact on their aerodynamic profile.
Swimming: Overcoming Water Resistance
While not air resistance per se, water resistance presents a similar challenge to swimmers. Reducing drag is critical for maximizing speed and efficiency in the water.
Shaving body hair, wearing specialized swimsuits, and adopting streamlined body positions are all strategies used by swimmers to minimize water resistance. The science of hydrodynamics plays a vital role in understanding and optimizing swimming techniques.
Skiing: The Need for Speed
In skiing, particularly downhill skiing, air resistance is a major factor affecting speed. Skiers adopt aerodynamic tuck positions to minimize drag and maximize their velocity.
Specialized suits and helmets are designed to reduce air resistance, and skiers often train in wind tunnels to refine their body positions. Every fraction of a second counts, making aerodynamic optimization a priority.
Honoring the Pioneers: Isaac Newton's Legacy
Air resistance, a force often overlooked, is fundamental to understanding the motion of objects through the atmosphere. It's the invisible hand that slows a skydiver's descent, shapes the design of a high-speed train, and determines the trajectory of a baseball. This opposing force dramatically influences everything from the speed of a falling leaf to the fuel efficiency of an aircraft. Yet, to truly appreciate the complexities of air resistance, we must first acknowledge the bedrock upon which our understanding rests: the work of Sir Isaac Newton.
The Newtonian Foundation of Force
Newton's Principia Mathematica, published in 1687, revolutionized our understanding of the physical world. Central to this revolution were his three laws of motion, which laid the groundwork for all subsequent studies of force and motion, including the nuanced field of air resistance.
His First Law, the law of inertia, establishes that an object in motion will stay in motion with the same speed and in the same direction unless acted upon by a force. Air resistance, of course, is that force, constantly working to decelerate moving objects within our atmosphere.
The Second Law, perhaps the most influential, mathematically defines the relationship between force, mass, and acceleration (F=ma). This simple equation provides the basis for quantifying the effects of air resistance. It demonstrates that the greater the force of air resistance acting on an object, the lower its acceleration will be.
Newton's Third Law, stating that for every action, there is an equal and opposite reaction, is also subtly present in the phenomenon of air resistance.
The moving object exerts a force on the air, and in turn, the air exerts an equal and opposite force back on the object.
Beyond Gravity: Recognizing Resistive Forces
While Newton's initial focus was primarily on gravity, his laws inherently provided a framework for understanding all forces. He established the conceptual tools necessary to analyze any interaction that changes an object's state of motion.
This conceptual shift was crucial.
It allowed scientists to move beyond simply describing motion to understanding the underlying causes of motion, including the resistive forces like air resistance.
The Unfolding Scientific Tapestry
It’s important to note that Newton’s work wasn't the final word, but rather the cornerstone of a continuing scientific investigation. Subsequent scientists built upon his foundation, developing more sophisticated models to describe the complexities of fluid dynamics and aerodynamics.
However, none of this later progress would have been possible without Newton's initial groundbreaking contributions.
He provided the fundamental laws and conceptual framework that enabled future generations of physicists and engineers to unravel the mysteries of air resistance and its profound impact on the world around us.
A Debt of Gratitude
In conclusion, while the study of air resistance involves intricate details of fluid dynamics, pressure gradients, and airflow patterns, we must always remember its origins in the work of Isaac Newton.
His laws of motion are the essential foundation upon which all our knowledge of force and motion is built. Acknowledging his legacy is not simply a historical formality.
It is a reminder of the power of fundamental scientific principles to unlock the secrets of the universe and improve our understanding of the world we inhabit. It's also a call to continue building upon this foundation, pushing the boundaries of knowledge, and seeking a deeper comprehension of the forces that shape our reality.
Video: Is Air Resistance a Contact Force? Science
FAQs: Is Air Resistance a Contact Force?
How does air resistance work, and why does it matter?
Air resistance is the force exerted by air on objects moving through it. It arises because air molecules collide with the object's surface. This matters because it opposes motion, slowing things down. So, is air resistance a contact force? Yes, it is.
What makes something a contact force?
A contact force requires direct physical touching between two objects. Think of pushing a box or friction between your shoes and the ground. The key characteristic is that force needs a physical link. In that sense, is air resistance a contact force? It absolutely is.
Is air resistance a contact force, even though we can't "see" it touching?
Yes, even though it's invisible, air resistance is a contact force. The individual collisions of countless air molecules against an object's surface are what create it. These tiny, constant impacts transmit force, making it contact. Is air resistance a contact force in this case? The answer is yes.
How is air resistance different from other types of forces, like gravity?
Air resistance requires direct physical contact, whereas gravity is a field force. Gravity acts at a distance without touching. Since air resistance arises from the air molecules colliding with the object itself, is air resistance a contact force? Yes, because it relies on direct interaction.
So, is air resistance a contact force? The answer, as we've explored, is a resounding yes! Hopefully, this has cleared up any confusion and given you a better understanding of how air interacts with moving objects. Now, go forth and impress your friends with your newfound physics knowledge!